the Creative Commons Attribution 4.0 License.
the Creative Commons Attribution 4.0 License.
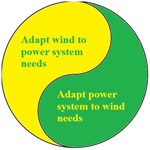
Grand challenges of wind energy science – meeting the needs and services of the power system
Mark O'Malley
Hannele Holttinen
Nicolaos Cutululis
Til Kristian Vrana
Jennifer King
Vahan Gevorgian
Xiongfei Wang
Fatemeh Rajaei-Najafabadi
Andreas Hadjileonidas
The share of wind power in power systems is increasing dramatically, and this is happening in parallel with increased penetration of solar photovoltaics, storage, other inverter-based technologies, and electrification of other sectors. Recognising the fundamental objective of power systems, maintaining supply–demand balance reliably at the lowest cost, and integrating all these technologies are significant research challenges that are driving radical changes to planning and operations of power systems globally. In this changing environment, wind power can maximise its long-term value to the power system by balancing the needs it imposes on the power system with its contribution to addressing these needs with services. A needs and services paradigm is adopted here to highlight these research challenges, which should also be guided by a balanced approach, concentrating on its advantages over competitors. The research challenges within the wind technology itself are many and varied, with control and coordination internally being a focal point in parallel with a strong recommendation for a holistic approach targeted at where wind has an advantage over its competitors and in coordination with research into other technologies such as storage, power electronics, and power systems.
- Article
(3790 KB) - Full-text XML
- BibTeX
- EndNote
It is widely accepted that the near-term focus for rapid and substantial emissions reductions in the energy system is the decarbonisation of electricity and the electrification of other sectors of the economy (IEA, 2022). Wind and solar photovoltaics (PV1), compared to the alternatives, i.e. costs and maturity of nuclear energy and/or carbon capture and storage, are dominating newly installed electricity capacity globally (EIA, 2022; IEA, 2022; Lazard, 2023; REN21, 2023). This dramatic increase in wind and solar PV has prompted a recent review of the status of these two key technologies to determine their long-term research challenges (Haegel et al., 2019; Veers et al., 2019, 2022; EAWE, 2023). These papers arose out of an International Energy Agency (IEA) initiative (Dykes et al., 2019). They identified a common research challenge, grid integration2, the all-important task of ensuring that with increased penetration of wind and solar PV in power systems,3 the primary objective of maintaining supply–demand balance reliably and at the lowest cost is met (O'Malley, 2011).
Increases in wind, solar PV, and electrification of other energy carriers (e.g. hydrogen) and sectors (e.g. industry) are also driving a rapid increase in other complementary technologies. These include flexible demand, electric vehicles, and sector coupling, which more broadly includes heat pumps and storage (batteries, power to heat, power to hydrogen, etc.), as well as changes to the grid infrastructure including offshore grids and increased high-voltage direct current (HVDC) grids (National Academies of Sciences, Engineering, and Medicine, 2021; Pineda and Vannoorenberghe, 2023). Many of these technologies come with their own integration challenges and opportunities (Matevosyan et al., 2021). All these simultaneous changes lead to a high-dimensional situation where meeting the primary objective is not a simple problem of wind integration but rather a multidimensional energy systems integration challenge (O'Malley et al., 2016).
Wind power in this rapidly changing environment needs to adapt competitively where it can by reducing any negative impacts and by contributing positively to meeting the power system's primary objective. That is, wind power can become part of the solution instead of being part of the problem. Instantaneous penetration levels of 100 % wind have already been reached in some regions (e.g. Denmark) but have not been reached in a country-scale synchronous power system4 (Söder et al., 2020). At current rates of deployment and considering the stated targets, instantaneous penetration of 100 % wind in synchronous power systems will be a common event in coming years, with 100 % wind energy penetration being approached in the coming decades, e.g. in Ireland (Denholm et al., 2021; Government of Ireland, 2021). Over the past few years, there have been many papers on the topic of 100 % renewables, which typically do not delve into the power system challenges and are therefore of limited utility. The reader is cautiously directed to many papers on the topic; see, for example, Breyer et al. (2022).
Maintaining supply–demand balance reliably and at the lowest cost in high wind penetration areas (and solar PV) comes with significant integration challenges (Hodge et al., 2020; Holttinen et al., 2020; Denholm et al., 2021). To describe the opportunities for wind power in this integration challenge, a systematic framework that coincides with planning and operational and market practices is required to define a set of power system needs and power system services (Chaudhuri et al., 2024). Historically the needs and services paradigm was inherently embedded in the vertically integrated utility concept and was not explicitly stated; more recently it formed the basis of electricity markets (Schweppe et al., 2013; Kirschen and Strbac, 2018). Most fossil fuel and nuclear and hydro generation plants are interfaced with the grid using synchronous machines (SMs)5 and are the main providers of most of the power system services. When these SMs are in sync with one another, they form a synchronous power system. SMs have been well known and understood for decades, and they are at the heart of the planning and operations of power systems (Glover et al., 2012). Increased wind power and other technologies are changing existing power systems and therefore the needs, and if they replace other technologies, e.g. SMs, there will be a reduction in the supply of some services. This requires wind power and all other technologies connected to the grid to meet these changing needs with services (EirGrid, 2023).
The IEA initiative (Dykes et al., 2019) has evolved, and this paper is part of the “Grand Challenges of Wind Energy” publication series (hereafter referred to as Grand Challenges; Veers et al., 2022) focussed on research challenges for wind technology that address the integration challenges. By their nature, integration challenges can be technological, market based, policy related, standards related, legal, and/or societal (Ahmed et al., 2020; Diógenes et al., 2020; Zhou and Solomon, 2020; Susskind et al., 2022; Kirkegaard et al., 2023). Here we focus only on research challenges related to the wind technology. The remainder of this paper is organised as follows. Section 2 introduces the integration of wind into power systems and into the services paradigm, which is the basis of the assessment of the research challenges that are detailed in Sects. 3–6. Section 7 is a discussion with some recommendations, and Sect. 8 concludes.
From an integration perspective, the characteristics of wind and solar PV (ESIG, 2019; Denholm et al., 2021) fall into two broad areas:
-
the operational variability, uncertainty, and distributed nature of the primary energy source that impacts the economics and reliability of the power system as penetration levels increase and
-
the interface of most of the wind and solar PV with the grid, not by SMs but by power electronic inverters/converters6 that are highly controllable, non-synchronous,7 and have limited overloading capabilities that mainly impact the reliability of the power system.
The operational variability, uncertainty, and distributed nature of the primary energy resource is a major difference in wind and solar PV when compared to other primary sources such as fossil fuel, nuclear generation, and hydro with pondage (Bird et al., 2013). It is not the case that these other primary sources are not variable, uncertain, and distributed; they are, but the characteristics are different. These differences are all related to the fact that these primary energy sources can all be easily stored; hence, the variability and uncertainty can be buffered. Fossil and nuclear fuel can also be transported to take advantage of economies of scale at a centralised location. In the case of wind and solar PV, the primary energy source cannot be stored and hence the generation is more distributed. The full variability and uncertainty need to be balanced internally by wind and solar PV by curtailing or by other parts of the power system such as other generation, demand, or storage, which are described in the literature as the need for flexibility (Lannoye et al., 2012). Curtailing wind and solar PV (or storing it) need the right economic incentives and can therefore be part of the solution providing flexibility instead of solely demanding flexibility from the system (Morales-España et al., 2021).
In the early days of the wind industry (1980s to 2000s), from a reliability point of view the “do-no-harm” philosophy was adopted; i.e. if there were problems in the power system, the wind power would invariably just disconnect so as not to cause any further reliability issues (Christensen, 2010; Lauby et al., 2011; Zavadil et al., 2011). Therefore, wind power did not impose any additional needs on the power system, and the only service wind provided was energy that was dependent on the wind availability on the day, with little or no operational planning with a forecast to account for wind variability and uncertainty. At that time, driving down the levelised cost of energy (LCOE) was the major objective of the wind industry as it still needed heavy subsidies. This modality of operation was pervasive until around 1–2 decades ago when wind power started to represent a significant portion of energy provision in some power systems. Inevitably some needs driven by wind were recognised, and additionally some services were required from and/or incentivised by wind power such as forecasting, fault ride through, active power regulation for frequency control, and the local voltage control that has been adopted in several power systems (Mohseni and Islam, 2012; EirGrid, 2019; IRENA, 2022).
During this period, the concept of calculating the so-called integration costs was mooted (Smith et al., 2007; Ueckerdt et al., 2013). There is wide literature in this area even though it can be easily shown that calculating the integration cost of a specific technology in an absolute sense, e.g. wind, is impossible and remains a controversial subject (Müller et al., 2018). Relative integration cost can be calculated by comparing two different portfolios, i.e. two power systems with different portfolios of generation, and other technologies that serve the same demand with equal reliability can have their costs compared (EirGrid, 2008; Holttinen et al., 2019). The portfolio cost is highly system dependent and can increase rapidly as penetration levels of wind increase (Cochran et al., 2014). For example, in a power system that is inherently flexible, the cost of operational variability and uncertainty while maintaining reliability may be negligible up to a point where the flexibility saturates; portfolio costs can then rise rapidly (Fig. 1). These costs can and do occur throughout the power system and can range from a direct part of the cost of the wind to costs elsewhere in the power system, but as stated above, it is impossible to allocate these costs to a specific technology.
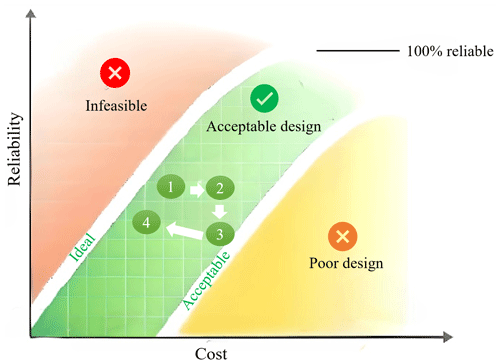
Figure 1Cost–reliability plane for power system design. The ideal design boundary inherently maximises the benefit by minimising the cost, subject to a required level of reliability. Above this ideal there is an infeasible region, and there is also an acceptable design boundary below which the power system design is deemed to be poor and not acceptable. Between the ideal and acceptable boundaries is the acceptable design region where the design is deemed to meet the primary objective. The progression from 1 through 4 represents a possible trajectory that is reflective of the evolution of designs as VRE penetration increases. From 1 to 2 there is a cost decline, e.g. having to do with VRE competitive economics; from 2 to 3 is a decline in reliability due to the impact of increasing VRE (e.g. lower inertial response); at 3 a new service (e.g. fast frequency response) is introduced to prevent moving into an unacceptable region; and this moves the power system to 4 where it is close to the ideal boundary.
2.1 Wind technology for power system integration
Four distinct wind turbine generator technology classifications exist (Fig. 2). Type I and II dominated the early years but are no longer deployed at scale. Power electronics began appearing in wind turbines around 20 years ago with the development of Type III wind turbines that employ a wound rotor induction generator with a four-quadrant power converter. This addition affords the capacity to regulate rotor circuit currents, resulting in an expanded operational range compared to Types I and II. Type III, also known as a doubly fed induction generator (DFIG), is characterised by flux vector control, effectively decoupling active and reactive power components to optimise output power. DFIGs have many advantages over Types I and II including lower cost, lower weights, and the ability to control both active and reactive power (Ackermann, 2012). Type IV involves either a synchronous or an induction generator connected to a full-scale back-to-back frequency converter that isolates electrical generator dynamics from the grid (Singh and Santoso, 2011). Variable speed wind turbines (Types III and IV) are now the dominant technology. Type III is the most installed onshore, while Type IV dominates the offshore market (WindEurope, 2023). The Type IV wind turbine has additional advantages such as optimised operation over all wind speeds, full control of active and reactive power production, augmented transient handling capability, and improved power quality (Chen et al., 2009).
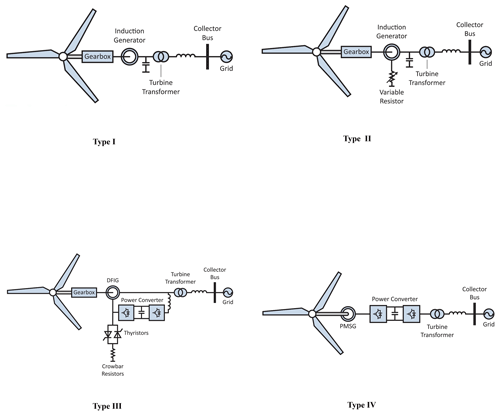
Figure 2The four types of wind turbine generator technology. Type I: squirrel cage induction generator. Type II: squirrel cage wound rotor induction generator with external rotor resistance. Type III: double-fed non-synchronous generator. Type IV: full power converter generator (Osman et al., 2018, used with permission).
The power electronic inverters/converters that connect wind and solar PV to the grid have fundamentally different characteristics than SMs with respect to their ability to, e.g. maintain the frequency of the power system within stable limits. Other new technologies that are similarly interfaced with the grid by power electronics and may add to these challenges include batteries, high-voltage DC (HVDC), etc. Inverter-based resources (IBRs) are used to collectively describe these technologies, which are interfaced to the grid by power electronic inverters/converters (Lin et al., 2022). The penetration levels of wind and solar PV are now so high in many power systems that they are beginning to replace fossil fuel generation (and even nuclear generation in some cases) in planning and operational time frames (Chaudhuri et al., 2024). Therefore, there is a trend towards the disappearance of SMs and their replacement with IBRs. This trend has consequences: a change in the needs and the supply of services, which may in the future be supplied by IBRs including wind. Power systems that approach 100 % renewable generation will face the dilemma of keeping SMs with significant cost implications to provide services or of relying on wind and/or other IBR-based technologies (solar PV, battery, etc.) to provide the services (Hodge et al., 2020). Simply keeping SMs is not in itself a perfect solution since they may not be at the correct location or may be too expensive (Appleby and Rositano, 2019).
Of note is the ability of IBRs (via the controls) to not only operate in grid-following mode (that is, other technologies, typically SMs in the power system, set the frequency and voltage for them to follow) but also in grid-forming mode (with the capability of setting and maintaining frequency and voltage), and they can also combine characteristics of both (Kroposki et al., 2017). This is an extremely active research field in the power systems and power electronics domains (Ackermann et al., 2017; Kroposki et al., 2017). For example, there is significant research activity to try and establish what is the best ratio of grid-forming and grid-following inverters in an IBR-dominated system, and the current estimates show the grid-forming penetration at around 20 %–30 % (Matevosyan et al., 2021). While the fundamental principles of IBR controls are a research topic regardless of the host technology, the implementation in wind turbines will need to be adjusted or tailored to their characteristics (Veers et al., 2023). Similarly for other host technologies, power-electronics-related solutions will be developed for IBRs, such as solar PV and battery storage, and this blurring of the boundaries between the technologies is to be expected as the power system becomes much more integrated and the wind technologies become more heterogeneous.
Another example of this heterogeneity is the research challenges for planning and operating offshore grids (Cutululis et al., 2021; Tande et al., 2022). These include optimising the stepwise offshore grid build-out, including offshore energy hubs and hybrid AC/DC grids, considering uncertainties and the long lifetime of the infrastructure, future connected wind capacity, and hydrogen demand. Other additional considerations are dynamic electrical cables for floating wind power plants and either floating or subsea substations to connect the wind power plant to the offshore transmission grid, as well as subsea collection systems for grid connection of large floating wind power plants.
Hybrids are another good example of heterogeneity, e.g. wind power combined with storage and solar PV, which can help to lower the impact of variability and uncertainty and hence reduce the need for some services (Stenclik et al., 2022). In hybrid plants, for example, the addition of storage across timescales from short duration to even long duration storage in future and the addition of solar PV, which may be a complementary resource to wind, will help increase the plant's ability to provide services to meet the needs of the power system (Nema et al., 2009; Stenclik et al., 2022). It is important to note that the advantages of combining the technologies come from shared transmission capacity, the quantity of power electronics, and controls, which can therefore maintain the performance and the overall quality of the services provided to the power system at a reduced cost. There are also regulatory, subsidy, commercial, and market advantages that can be very system specific. There are no purely synergistic technical advantages inherent in hybrids, and from a services perspective, there may be limitations imposed by constraining the technologies to act in a coordinated manner (Stenclik et al., 2022; Kemp et al., 2023).
2.2 Future needs and services: an opportunity for wind power
For the power system to meet its primary objective, wind and other technologies need to adapt to the power system, and/or the rest of the power system needs to adapt to them in much the same way as occurred historically with SMs (Fig. 3). Wind no longer must be accommodated, but rather the power system needs to enable its increased penetration. The end destination in this process is far from clear but will be heavily influenced by research and innovation in power systems and the technologies that make up the power system including wind (Veers et al., 2019). In this changing environment, power system needs and services should be assessed to ensure power system reliability at the lowest cost and/or to avoid providing services that are no longer needed, which can be costly. Resources, the policy environment, and the stage of development are different across the world, and there is and will be a wide variety of power systems with distinct characteristics. While most power system needs and services will share a lot in common, some of them will be system specific.
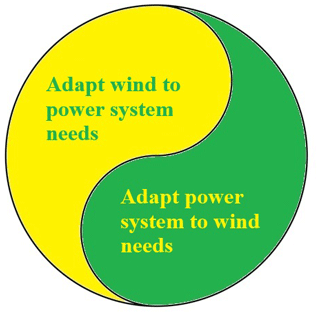
Figure 3Wind adapting to the power system, and the power system adapting to wind – a pathway to a cost-effective, reliable power system.
The required services can be found in different parts of the power system, but here we focus on wind-based solutions (Fig. 4) that require significant research in the coming decades and that may have the potential to be competitive against other sources of these services. For wind this translates into a multiscale research and design challenge at the individual turbine, plant, or hybrid level, with mechanical-, electrical-, and/or control-centric solutions to the provision of services and a techno-economic comparison with other alternative sources for these services to meet the power system needs (Fig. 4). For other technologies such as solar PV and batteries, this translates similarly.
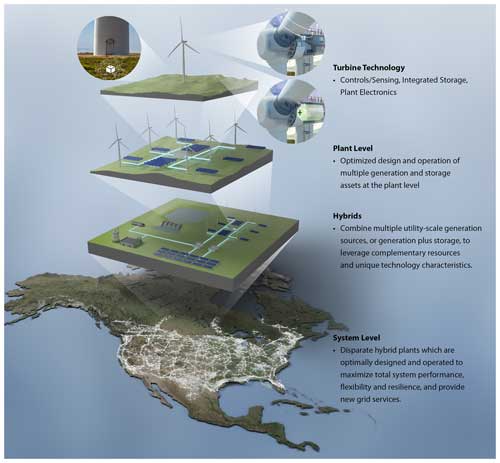
Figure 4Wind turbine to plant to hybrid to power system level and the provision of services to meet the power system needs.
Procurement of services to meet the needs can range from a mandated capability (i.e. interconnection requirements/grid codes) to a capability that is paid for in a monopoly or to a formal competitive market scenario and all the variations in between. In some cases, the need may be inherently met with no scarcity and hence no need for a mandate or incentives (Ela et al., 2019, 2021). This variation is evident in the range of services that exists in many of the electricity markets across the world (Rebours et al., 2007a, b). Procurement mechanisms are evolving in parallel to incentivise the services to meet the needs in an optimal manner (Hobbs et al., 2022). It is important that the wind industry is incentivised by market signals, grid codes, etc. and through design and innovation to mitigate needs and/or provide competitive power system services. The wind industry needs the right incentives so that wind technology evolves to maximise its value to the system rather than solely maximising its power output. With wind and solar PV having near-zero marginal cost, this is also challenging electricity market and policy design (Neuhoff et al., 2023). Innovations in response to wind such as societal acceptance and lifestyle adaptations to energy availability are also changing (Schuitema et al., 2018; Steg et al., 2018). These non-technical challenges are not addressed in this paper.
There is no ideal way of cleanly defining needs and services, as the power system is a highly integrated continuum of overlapping, interacting technical characteristics from a wide range of technologies all acting in unison to maintain supply–demand balance reliably and at the lowest cost. Needs are not necessarily met by individual services but by combinations, while other characteristics and functionalities enhance a service that meets a need or reduces a need. Furthermore, moving into the future the needs themselves are uncertain, as they are subject to evolving research in the power systems community, the technical characteristics of the IBRs and other new technologies, and the reliability requirements that are also evolving, in addition to the technological methods that can actively maintain security standards (Hedman et al., 2011; Bialek et al., 2021; O'Malley, 2022). A good example of this evolution is the recent update to the classification of stability needs in power systems, which is driven almost exclusively by IBRs (Hatziargyriou et al., 2020). Here we adopt the work of the Global Power System Transformation Consortium (G-PST) and the proposed structure of eight distinct needs that broadly include the stability needs identified by Hatziargyriou et al. (2020), i.e. energy and capacity, and the six technical needs further grouped into frequency and voltage control, synchronisation and damping, and protection and restoration (Fig. 5).
In the following four sections, wind technology is assessed with respect to meeting these needs in electricity systems driven by increasing penetration of wind and other technologies. The sections are organised logically around the grouping of the services indicated in Fig. 5.
Energy and capacity are the two most basic power system needs, as supply–demand balance cannot be met if there is not enough energy or if at any time, instance, or location there is not enough available capacity (generation, transmission grid, etc.). In many power systems, wind is providing a significant proportion of the energy needs. For example, in Denmark wind accounts for over 50 % of the electricity demand on average, more than 100 % in some instances (Holttinen, 2023), and several other jurisdictions are targeting 80 % to 100 % annual energy from wind and solar PV, with wind being dominant in some cases such as in Ireland (SEAI, 2023). In many respects, the energy need and/or service is the primary focus of the other papers in this Grand Challenges series, which focus on lowering their costs and increasing their lifetimes, reliability, and performance (Veers et al., 2022, 2023).
Resource adequacy, the ability to meet the demand, has traditionally been about capacity and is equivalent to having the generating capacity and transmission to serve the demand at every point in time and at every location (Schweppe et al., 2013). It could traditionally be approximated as the ability to meet peak demand, but wind and solar PV is changing this and moving the critical time to other periods of low wind and/or solar PV, e.g. peak aggregate net demand (demand minus wind and solar PV). This is undermining some of the calculation methodologies that were based on assumptions that may no longer be valid and may need to evolve (Stenclik et al., 2021). Having abundant volumes of energy at times when demand from the consumer is low is of minimal value and vice versa. Therefore, when the timing of energy from a technology correlates with demand, this increases the capacity value of a technology and is fundamental to maintaining the reliability of the power system (Keane et al., 2011). SMs with readily storable primary energy sources (e.g. fossil fuels, nuclear power, and hydro with pondage8) are dispatchable and can therefore be available at peak times and have capacity values close to the maximum of unity (the minimum is zero). However, the capacity value of wind (and solar PV) is relatively low compared to SMs, ranging between 10 % and 35 % (Denholm et al., 2019; Holttinen et al., 2021).
The capacity value of wind reduces with wind penetration due to the correlation effect, and it varies from year to year depending on weather patterns (Hasche et al., 2010; Cradden et al., 2017). Therefore, with increasing wind (and solar PV) penetration and the displacement of SMs, the contribution of wind declines, leading to an adequacy deficit that economically constitutes the single biggest challenge to very high penetration of wind and solar PV (ESIG, 2019). Any design of wind power technology that improves generation at lower wind speeds and increases the number of running hours should improve the capacity value of wind. For example, low-wind designs with larger rotors may in the correct circumstances increase capacity value (Dalla Riva et al., 2017; Wiser et al., 2020; Swisher et al., 2022). In general, for best impact on capacity value, diversification of turbine types is key as it reduces the correlation between their energy output; for example, transmission capacity enables this diversification, as does mixing of onshore and offshore wind (EPRI, 2022a). An extreme version of this and a long-term research topic is airborne wind power plants that can harness the steadier wind resources at a higher altitude than regular wind turbines, with potentially far-higher-capacity values, but this technology faces very significant research challenges (Kolar et al., 2013; Cherubini et al., 2015; Bechtle et al., 2019).
As stated above, needs and services are overlapping and interacting. A good example of this overlap is energy and capacity, as is evidenced by the debate around energy-only markets (where capacity is incentivised by high energy prices during periods of scarcity) and capacity markets (where capacity is directly rewarded; Hogan, 2005). The focus is now shifting from minimising LCOE towards maximising the value of wind, which effectively captures all the services (Denny and O'Malley, 2007; Dykes et al., 2020; Loth et al., 2022). It no longer matters that the cheapest possible electrons are being put into the grid (as energy), but what matters is when and where those electrons are put into the grid (requiring the energy and capacity), adding additional value to the system. Typically, energy and capacity are the most valuable services, but there are indications that as we approach higher penetration of wind and solar PV, the more technical services covered below and/or developed to meet future needs could see their relative values increase (Ela et al., 2017). Therefore, with the changing needs and services, there is a need to strike a balance between maximising the value of energy and capacity without potentially undermining the cost effectiveness or ability to provide the technical services, which could unduly increase the portfolio cost and/or degrade the reliability of the power system (Fig. 1). A good example of this can be found in this series of Grand Challenges papers where plant controls can increase the energy yield of wind power plants, reducing wake losses and loading of components, and are also the way that wind can provide more cost-effective power system services (Meyers et al., 2022; Veers et al., 2023). Energy management systems that seamlessly provide energy and other services optimally are key to provide profitability of wind plants to owner–operators while maximising value to the power system when needed (Van Dijk et al., 2017). This is an ongoing research topic (IEA, 2023b). These energy management systems will need forecasting to determine the expected energy and other power system services from wind and to do so in a coordinated manner; e.g. if wind is curtailed, the wind capability after curtailment needs to be estimated (Göçmen et al., 2018).
The use of wind-based hybrids together with solar resources (the less correlated with wind the better) can maximise grid capacity and at the same time reduce the need to build out the grid and can be beneficial. This approach can result in higher aggregated capacity factors9 (average power output with respect to maximum rated output) even if the shared point of interconnection can reduce the capacity factor of each individual technology (EPRI, 2022b). Hybrids with storage can make a significant contribution to the adequacy need and are an active area of research and deployment (Murphy et al., 2021). The storage device can be used across timescales to move the energy from less into more valuable time periods. This is true regardless of whether the storage device is co-located with the wind or not; however, directly coupling wind with solar PV and storage can bring down costs, especially when network constraints exist (Jorgenson et al., 2018; Mallapragada et al., 2020). Non-hybrid solutions where wind and storage are not subject to the same constraints may be more beneficial (EPRI, 2022b).
The continuing development of power system coupling to other energy sectors gives rise to new opportunities for using the energy generated from wind (Van Nuffel et al., 2018). Power-to-X solutions, producing hydrogen and its derivatives, e-fuels like ammonium and methanol, and synthetic gases, may in future occur locally at wind power plants if the grid is congested and may provide storage for wind (Singlitico et al., 2020).
Future weather-dependent power and energy systems require more data and improved tools, in which wind energy needs to be well represented. Wind and solar PV impacts on power systems give rise to considerations of energy adequacy (not enough energy resources for generation even if installed capacity is adequate) and the adequacy of other services (EPRI, 2022b). In the future as not only power generation but also demand will be increasingly weather-dependent due to electric heating and cooling, correlated events caused by common weather patterns need to be considered more carefully when determining resource adequacy for the planning of energy systems (Novacheck et al., 2021; Stenclik et al., 2021). Different weather authorities are working on improving seasonal weather forecasts. The focus of their work is calculating the forecast uncertainty using probabilistic ensemble forecasts instead of improving the expectation value of the forecast (Leutbecher and Palmer, 2008). Seasonal demand shifting of industrial loads also has potential but is nascent in its research and development (Yang et al., 2020).
Research to drive down LCOE is now being overtaken by the need to maximise the value of the wind with respect to evolving energy and capacity needs and with respect to other services that are themselves evolving (Tables 1–7). To achieve this, the research focus is being pushed outside the wind technology and towards storage technologies and, e.g. power-to-X, which needs to work in a coordinated manner with the core wind technology. The balance between these becomes a higher-dimensional co-optimisation problem, in which all potential value streams are accounted for including the six more technical needs/services of the power system. These six technical needs/services focus primarily on the reliability of the power system, starting with the power system's two most important attributes, frequency and voltage (Tables 2 and 3).
The primary technical attributes of a power system are its frequency and voltage. The frequency is a global attribute that should be kept within preferred limits around a nominal value (typically 50 or 60 Hz) throughout the grid. The voltage is a local attribute that should be kept close to constant, which is different in different locations (ranging from a few hundred volts to just over one million volts). Controlling the frequency and voltage around their nominal values is critical to maintain the reliability of the power system.
4.1 Frequency control
Frequency control directly addresses flexibility. Too little supply or too much demand reduces frequency and requires an increase in generation or a decrease in demand; too much supply or too little demand increases the frequency and requires a decrease in generation or increase in demand to maintain the frequency within its preferred limits. Frequency control will be impacted by both variability and uncertainty in aggregate net demand, as well as by the relative number of SMs and IBRs online. There is considerable beneficial smoothing of variability and predictability (in normalised metrics), but increased wind and solar PV will result in a more variable and less predictable net demand. This drives the need for frequency control and more flexibility resources throughout the power system. The power electronics and most importantly its control that interfaces wind with the grid typically decouples the mechanical inertia of the wind turbine from the grid. Inertial response from SMs is inherent, instantaneous, and impossible to prevent, and with large numbers of SMs (mainly synchronous generators) on a power system it was traditionally abundant, and there was no need to reward it (Eto et al., 2010; Muljadi et al., 2012). If SMs are replaced by IBRs, this will lead to a reduced inertial response, the frequency control need may not be met, and alternatives will need to be found (Doherty et al., 2005; Ela et al., 2013; NGESO, 2023). This inertia issue has received significant research attention recently, although in some smaller systems it has been central for several decades, emphasising the point that every system is different (Mullane and O'Malley, 2005, 2006; Doherty et al., 2005; Denholm et al., 2020). Larger systems with pockets of IBRs and a lack of SMs can also exhibit localised low-inertia-type behaviour during faults (Abdul Wahab and Mohamed, 2012; Badesa et al., 2021).
The timescales of variability, predictability, and inertial response are different. The variability and predictability result in a need for relatively slow frequency control, while the inertia issue results in the need for faster frequency control. Frequency control goes from very fast inertia-like response times (seconds and below), to fast frequency response (seconds), to primary frequency response or governor response (seconds to minutes), to regulation reserve or automatic generation control reserve, to slower flexibility reserve or load following (minutes and beyond) – eventually frequency control merges with the energy service (Ela et al., 2019). This highlights that these needs lie on a continuum and shows the overlapping nature of the services as, for example, faster frequency response reduces the need for inertial response (Delille et al., 2012).
Wind turbines can provide frequency control services and have done so for at least a decade (Ela et al., 2014). Wind turbines can be curtailed to decrease generation to decrease frequency, and when curtailed, wind can increase generation to increase frequency. Typically, wind turbines will control their pitch and torque to change their power output. These control mechanisms operate on the order of seconds, something that allows them to follow reference signals that operate in 4 s intervals, for example, which is the timescale of automatic generator control in many power systems (Bevrani and Hiyama, 2011). Therefore, wind can provide many aspects of frequency control services but may not be competitive because of the energy losses due to curtailment of wind. In some operational circumstances such as stability constraints and minimum generation constraints, wind may already be partly curtailed, making frequency control more competitive (Denholm et al., 2019). One way of avoiding the curtailment of wind when providing frequency control in an upward direction is to use the energy stored in the rotating blades that can provide frequency control by slowing down the blades without any pre-curtailment of wind energy. With this method, a wind turbine can provide a power boost of 5 %–10 % of its rated power. While this effect may only be temporary (as there is a limit to how much you can slow down the blades), it can work together with other mechanisms to help restore frequency to a stable level (Bonfiglio et al., 2018). Wind as part of a hybrid with storage can also provide frequency control services. One of the challenges to be addressed is the optimised control and operation of wind-based hybrids for frequency control support (Liu et al., 2019).
The fast controls of wind power plants (operation in seconds) have proven to give good compliance support in low-inertia operation (Ela et al., 2014; Denholm et al., 2021). For faster responses, some open issues remain, like the ability to measure, compute, and transmit the frequency signal to the wind turbine plant controller fast enough, with a response time on the scale of hundreds of milliseconds. Operating wind turbine power electronics in grid-forming mode would significantly decrease their reaction time and, to some extent, mitigate the challenge of measuring, computing, and transmitting the frequency change. The enhanced static synchronous compensator (E-STATCOM) that integrates supercapacitor and grid-forming control emerges as promising technology for furnishing wind farms with inertial power response (Zhao et al., 2023).
While initial field tests show the viability of such operation, they also highlight challenges, with the most pronounced one being the limited energy stored in the wind turbine rotor, which, if not properly managed, can lead to the opposite effect, i.e. reducing the power in-feed to zero (Roscoe et al., 2021). Also, grid-forming operation of wind turbines will most likely have an impact on the drivetrain, and the known mechanical impacts on wind turbine drivetrains that need to be addressed can result in additional costs and reliability impacts that may not be justified (Girsang et al., 2014; Gloe et al., 2021; Zhang et al., 2021; Chen et al., 2022; Nguyen et al., 2022). Under certain grid disturbances, such as phase angle jumps, active power oscillations may be induced within grid-forming wind turbines, which tend to propagate to the drivetrain of wind turbines and even trigger torsional vibrations, degrading the lifetime and reliability of mechanical components (Avazov et al., 2022; Lu et al., 2022). The recent work in Roscoe et al. (2020) has shown that without additional energy storage, grid-forming wind turbines have limited power responses under some unscheduled frequency disturbances of power grids. This highlights that the provision of services can have an impact on the cost and reliability of the wind technology and its performance. Another relevant example is when a turbine provides frequency control and changes its pitch and/or torque, which has implications for its wake that propagate downstream and impact downstream turbines. These impacts can be modelled using wake models and can affect the performance of downstream turbines if not considered properly (Houck, 2022; Meyers et al., 2022). While most studies assume that wind turbines are capable of accurately providing a short-term increase in their active power production, they usually employ very simplified representations of the flow; e.g. turbulence is not represented, even if it can have a large impact on the ability of the wind turbine to provide that extra active power (Veers et al., 2023). The energy contained in the airflow field generally supports the provision of the desired short-term increase, as a power increase from the curtailed state to above available power is possible (until the resulting wake propagates to the adjacent turbines), but the implications for service provision have not yet been investigated in detail. Plant control and flow control can also take advantage of wake steering to distribute curtailments in a smarter way and reduce losses when providing services. Wake steering happens on the timescale of minutes, so this is not as useful for the fast-response services.
An important part of frequency control is the ability to maintain the desired level of response in accordance with the service. Accurate wind power predictions are essential to offer such services reliably. Overestimates or underestimates of energy and/or frequency control capability will result in economic loss through curtailment and/or penalties. The wind industry can facilitate forecasting by the development of further systems and meteorological measurements at the wind turbine and plant level and by providing these measurements in real-time to weather services and forecast providers (Lin and Liu, 2020).
One research area that still needs to be addressed is the coordination of plants in a region. Currently, when frequency control services are required from wind plants, multiple wind plants respond. Similarly, as with SMs, this can create an oscillatory effect if the collective response is too strong and not properly designed/tuned. The impact of locational delivery of frequency response suggests that depending on where frequency response is injected in the network, it could have a positive or negative impact (e.g. additional congestion). As wind power plants are distributed, this can help to optimise the system-wide coordination of delivery of frequency response (Wu et al., 2018).
As offshore wind development will be combined with the development of offshore infrastructure based on HVDC converters, supplying frequency control, especially the inertial response with grid-forming control, will require proper coordination of control with the multiple converters in the loop: wind turbines, offshore HVDC, onshore HVDC, etc. (Gu et al., 2020). For HVDC-connected offshore wind power plants, the controllers of the multiple converters need to be properly coordinated (Glasdam et al., 2013; Sakamuri et al., 2017).
4.2 Voltage control
The effectiveness of voltage control is dependent on grid topology and the location of the plant. Heavily loaded transmission systems increase the risk of voltage instability. The main factors contributing to the long-term voltage instability and subsequent voltage collapse are (i) stressed power systems with high active and reactive power loading, (ii) inadequate reactive power resources, and (iii) load characteristics with respect to demand-side voltage (Van Cutsem and Vournas, 2007). The fluctuating nature of wind power can exacerbate the voltage instability, playing a significant role in decreasing the dynamic voltage stability margin of the system (Zhou et al., 2005).
Wind power plants can provide voltage control and have been doing so for many years. The impact of the distributed nature of the wind resources on voltage services is as follows: if the wind resource is on a distribution grid instead of a transmission grid, this can degrade its voltage control potential. Moreover, it is important to note that voltage control is a localised need. While wind power plants can be used to enhance the voltage control in some locations, there may be certain areas where the siting of wind turbines is not allowed.
Research is ongoing to better understand the limits and dynamic performance of reactive power provision from wind power plants and how to use and coordinate them in practice (Qiao et al., 2009; Ghosh et al., 2020). Grid-forming operation of wind turbines will enhance their ability to provide voltage control, an intrinsic characteristic of grid-forming operation. Research is still needed regarding optimising the use of all power electronic devices installed in gigawatt-scale offshore wind farms (like the static synchronous compensator, STATCOM, installed at the onshore connection point) to improve the dynamic range of reactive power capability. This reflects the highly integrated nature of research in this area, i.e. the multitude of options to solve the challenges inside and/or outside the wind plant (Veers et al., 2022).
Frequency and voltage control are services that wind is already providing, typically as part of a portfolio of other technologies such as SMs, and there are no specific long-term research challenges associated with wind providing voltage control (Table 3). As SMs disappear, the total reliance on wind and other IBR technologies will require additional innovations and coordination with other services, particularly frequency control (Table 2). The loss of inertial response will require very fast frequency response using, e.g. grid-forming controls, which will also improve voltage control. However, the proliferation of a multitude of IBRs in combination with reduced inertia will drive the need for services that address synchronisation and damping challenges (Tables 4 and 5), which are on the rise (Vittal et al., 2011) and are dealt with in the next section.
Frequency and voltage control, described above, are used to maintain frequency and voltage within given ranges around their nominal values. These ranges typically allow large deviations for short periods of time or for sudden events and have tighter ranges when the system is in steady state. However, in steady state and during events, there can be other consequences that can be detrimental to the reliability of the power system, such as oscillations in the power system that need to be damped and loss of synchronisation (Vittal et al., 2011; Wang and Blaabjerg, 2018).
5.1 Synchronisation and angle stability
As stated above, SMs are at the heart of the synchronous AC power system that currently dominates worldwide. If SMs decline and are replaced by IBRs including wind, this synchronous characteristic starts to diminish, and a loss of synchronism may occur, which can result in the disconnection of SMs and hence a catastrophic loss of generation and a subsequent blackout. To avoid a loss of synchronisation and instabilities induced by loss of torque due to large load angles between groups of SMs, typically due to a large and abrupt supply–demand imbalance event, an increase in the active power output proportional to rotor or voltage angle deviation is needed (Boldea, 2005). For wind, the mechanism of loss of synchronism can be significantly different from SMs, and it is highly dependent on the control method – grid forming or grid following. With more changes in the grid-following and grid-forming wind, simple fault ride through is not enough, and understanding the synchronisation stability and the impact of power electronics (current-limiting) and control are a challenge (Denis et al., 2018).
Synchronisation will be more critical in the transition phase when there will be significantly fewer SMs to provide this service. For power systems where there will be no SMs left, this synchronisation need will not exist. There may be a point in time in the transition with very few SMs active in the system, when the challenge will be temporarily very complex as the synchronous nature of the power system will give way to a non-synchronous system. Hence, this is a potentially transitory need, is linked to the much bigger debate on the fundamental nature of the power system going into the future, and includes the debate around the ratio of grid forming to grid following and needs and services with no SMs (Matevosyan et al., 2019; Bialek et al., 2021).
Synchronising service from wind is not provided today and is an emerging research area (Table 4). IBR-based wind power's role in this challenge could be to mimic an SM, but this may not be the best approach, and research efforts should be made to leverage the unique capabilities of the power electronics that interface the wind turbines with the grid (Pan et al., 2020; Liu and Wang, 2021). The research challenge is mainly associated with the availability of the extra active power needed during the re-synchronisation period. The extra active power may also be needed simultaneously for other services (frequency control, damping, etc.) to meet other needs, which makes the coordination of the services from wind highly complex (Denholm et al., 2019). One solution could be to use some of the overloading capabilities of wind turbines; however, this will depend on the duration and magnitude of the disturbance, and research is needed to understand the impact on expected performance and characteristics (Hansen et al., 2014; Moawwad et al., 2014; Altin et al., 2018). Another approach can involve the use of an energy source, either directly connected to the wind turbine plant or as part of a hybrid plant.
5.2 Damping
Power oscillations are generated because of interconnected power systems and power transfer operations. As modern power systems continue to become more and more interconnected to provide adequate power and access to capacity-constraint-based corridors, the propagation of these oscillations must be tackled for reliable and secure power system operation. Oscillations can be induced by (1) step changes in load, (2) a sudden change in generator output, (3) transmission line switching, (4) short circuits, or (5) changes in operating points. In existing power systems, these power oscillations are caused by SM rotor angle swings varying from 0.1 to 4 Hz (Rafique et al., 2022). Today, SMs are equipped with additional control loops, called power system stabilisers, which are used to enhance the damping of power system oscillations through excitation control.
With the increased share of IBRs like wind (and solar PV) with different characteristics – no natural inertia – dependence on control can lead to more frequent oscillations (Denholm et al., 2021). DFIG wind turbines can cause sub-synchronous oscillation (SSO) phenomena caused by the interactions between their controllers and series capacitor compensators (Xu et al., 2019). Similarly to synchronisation, the main challenge is the availability of active power and the coordination with other services.
There are some examples of power system oscillations that are not fully understood and do not originate from any clearly identifiable event, but there is evidence that they are related to increased IBR penetration from wind and solar PV (Cheng et al., 2022). One of the biggest challenges is trying to understand their source, something that also relates to the larger issue of having the correct models and tools to investigate these phenomena and solve them, typically by controller tuning (Miller et al., 2021).
There is a relatively large pool of literature related to power oscillation damping (POD) capabilities from wind turbines and plants (Domínguez-García et al., 2012). The oscillations can be damped by injecting either active or reactive power modulated at the terminal of wind plants (Zeni, 2015). It has been demonstrated through testing that wind power can modulate their active and/or reactive power output based on different control algorithms to provide damping services to the power system (Domínguez-García et al., 2012). It has also been demonstrated that DFIG wind turbines operating in grid-forming mode are less prone to SSO-type instabilities due to the different nature of their impedance characteristics (Shah and Gevorgian, 2020). While multiple POD-control approaches for wind turbines are presented in the literature, the capabilities have not been deployed or used in real-life projects.
As stated above for frequency control and synchronisation, wind turbines are quite capable of modulating their active or reactive power output. The challenge associated with this is mostly related to the availability of the extra active power needed if active power is chosen as the control variable. The coordination with other services is also a challenge, and hybrids with storage can alleviate this challenge and enhance this capability. A more challenging issue is the possible impact of the active power modulation on the wind turbine drivetrain since the low-frequency oscillations typically occur inside (or very close) to its natural frequencies (Ghasemi et al., 2013).
Providing synchronising power and/or damping is not fundamentally a challenge to wind turbines. The main research challenge will be related to the availability of the active power and in the coordination of the control with the other assets and services. While the power electronics may have some limitations, their controllability gives additional capabilities that SMs cannot provide and may enable better operation of the power system (Gonzalez-Longatt et al., 2021). These limitations are also a dominant theme of the final two power system needs that are discussed below, protection (Table 6) and restoration (Table 7), but the controllability may not bring a significant advantage.
Protection and restoration are extremely important needs for the power system. They protect human life and technologies including generation and transmission, protect transformers from severe damage and against cascading failures that may collapse the power system, and/or in the event of a collapse allow the power system to be restored.
6.1 Protection
Over-current protection is currently a power system need and SMs are a source of large currents that will flow during a fault. These currents are an essential triggering mechanism to activate the protection systems. The limited overload capabilities of power electronics in contrast to SMs and hence the lower fault current capability of wind turbines can undermine the traditional way of triggering the protection system. The challenge with protection also includes the fault current profile of IBRs including wind. It can vary between grid-following and grid-forming control and with the evolution of grid code requirement. The provision of negative-sequence current during asymmetrical faults can also challenge the efficacy of relays. If SMs retire and are replaced by IBRs, these needs for fault currents may not be met, and other protection methods may be required.
There are no known wind-based solutions for protection other than the fact that they can have some contribution to the fault current. The fault current limitation of IBRs can be addressed by oversizing the power electronics so they can produce higher currents during faults. However, this solution is very costly and will still not reach the same response that SMs can provide today (Bialek et al., 2021). DFIG wind turbines can provide much higher levels of fault current (6 times rated or higher) compared to full converter wind turbines (El-Naggar and Erlich, 2015). DFIGs could therefore help the situation but would need to be deployed at many locations on the grid. Ways to use the whole installed capacity of wind (and solar PV) that are much larger than SM capacity for providing the same fault current demand could be explored. Most of the time, wind power plants are generating less than the rated power and could run as STATCOMs when there is no wind available. However, the short circuit current will still have to – individually – be limited to 1.5–2 times the rated current at each connection point. Potentially significant changes are needed in protection methods once the SMs are all gone, pointing towards totally new approaches like using travelling waves (Wilches-Bernal et al., 2021).
Making alternative wind turbine generator technologies available may address some of the shortcomings of existing designs (Fig. 2). For example, Type V has been a concept for decades but is not commercially available. It consists of a variable-speed drive system linked to a torque converter, operating in tandem with an SM (Fig. 6). This SM can be seamlessly integrated into the grid through a circuit breaker, thereby conferring SM benefits to the grid (Camm et al., 2009). It may provide many benefits including fault current contribution and provision of inertia. These wind turbines are even capable of operating as synchronous condensers during periods of low/no wind and may be a significant part of the future power systems (Henderson, 2021; Henderson and Gevorgian, 2022). However, a significant drawback of this type is pole slipping, which can occur following a fault and result in either loss-of-load or loss-of-generation excitation. Additionally, the pulsating torques during pole slipping have the potential to inflict damage on the generator and gearbox if not addressed appropriately (Gevorgian et al., 2022).
6.2 Restoration
Restoration of the power system following a blackout is an important need that is rarely activated. To start a power system from a blackout situation requires both a primary energy resource and the ability to form the grid (grid forming). Wind, with its power–electronic interface, can provide grid-forming capability but can only provide an energy source when the wind is blowing. Although this is a rather new topic for wind power plants, studies and first demonstrations show that wind turbines equipped with power–electronic interfaces have self-sustaining islanding capabilities, meaning that – with a minimal energy source – they can start up, energise, and control their voltage and frequency (ScottishPower, 2023). Preliminary field tests show that wind turbines can operate in grid-forming mode and energise a substation (Roscoe et al., 2020), illustrating that wind can provide restoration services when wind is available (Pagnani et al., 2020; ScottishPower, 2023). However, as a first stage of the restoration process, there are certain control and design modification challenges (Achilles, 2018). It could help the power system if enough wind power plants equipped with black-start capabilities are located across the system in strategic locations including offshore, considering network energisation and nearby loads (Jain et al., 2020). Black-start-capable wind power allows implementing new bottom-up grid restoration strategies (as opposed to the conventional top-down approach by central thermal plants), helping to enhance grid resiliency and restoration times.
As the restoration services from wind power are nascent, there are multiple aspects that need to be addressed that from a needs and services perspective are like power system needs and services more broadly. Individual wind turbines can operate in grid-forming mode, but when multiple wind turbines in a plant are jointly controlling the voltage and frequency, often at a quite-low operation level (below 15 %–20 % power), synchronisation and unwanted interactions can occur (Lin et al., 2020; Henderson, 2023). Stability analysis methods and proper controller tuning methods need to be developed. The wide timescale and frequency coupling dynamics of power electronic converters tend to bring in harmonic instability in the form of resonances or abnormal harmonics in a wide frequency range (Wang and Blaabjerg, 2018), and the small signal stability of wind power plants operating in grid-forming mode for power system restoration (low-power operating point) needs to be investigated (Martínez-Turégano et al., 2020). The chances of large transients occurring during the restoration process are relatively high, as the system will be in a vulnerable state; hence, the performance of wind turbines operating in grid-forming and low-power operating points needs to be investigated thoroughly (Papadopoulos and Milanović, 2016; Jain et al., 2020). Hybrid with storage and wind and/or solar PV with grid-forming capability can self black start first, then energise transmission tie-lines, and finally participate in system restoration schemes (Bialek et al., 2021).
In times of blackouts, the provision of forecasts from external services may also break down. Power system operators may have to work with older forecasts that have worse quality. Research and development on probabilistic forecasting, providing reliable information about the available wind power, is essential for restoration concepts (NGESO, 2019).
Resilience, that is, the ability of a power system to transition and potentially survive large-scale events that may otherwise cause a blackout and/or ensure the survivability of key assets that will allow restoration, is an evolving area of interest to policy-makers and to the electricity industry more broadly (Panteli and Mancarella, 2015). It is still in its formation stage as a power system need and is likely to either add to existing needs and/or result in new needs and hence services. For example, improving the resilience of power systems against extreme weather events is one of the key concerns that designers and researchers are exploring, as the frequency of extreme weather events is on a rising trend due to climate change (Mahzarnia et al., 2020). These events have the potential to cause severe damage to power systems, leading to widespread blackouts that can have significant economic consequences (Bie et al., 2017). The role of wind energy in improving power system resilience involves research into turbines that can withstand high-speed winds during hurricanes and during the restoration process (GE, 2018; Simpkins, 2022).
There are some common themes arising from protection and restoration that are also reflected across other services, and this is discussed briefly in the final section along with some conclusions.
Wind can and does provide energy, capacity, frequency, and voltage control services, and these can be further improved and enhanced by research (Tables 1–3). There are nascent research efforts to investigate the potential of wind to provide synchronisation, damping, protection, and restoration services, and there is no fundamental reason why they cannot provide them; however, they may be neither competitive nor ground-breaking, and more investigation is required (Tables 4–7). This research needs to be done in the context of a fundamental change in power systems, driven by the replacement of SMs with wind and other IBRs. This transition is very dynamic and is presenting profound new research challenges to the objective of maintaining supply–demand balance reliably at the lowest cost. The simultaneous changes in increasing wind, solar PV, batteries, HVDC, etc. (and electrification) lead to a high-dimensional situation where the challenge is not a unidimensional wind integration challenge but a multidimensional energy systems integration challenge.
The lowest-cost solutions for resolving these research challenges can come from inside or outside the wind turbine plant, within the power system, and/or from the broader energy system. Here the focus has been on solutions in the form of services that come from within the wind technologies and those that are directly integrated with the wind technologies, e.g. power electronics and hybrids. This translates into a multiscale research and design challenge at the individual turbine and plant level, with mechanical-, electrical- and/or control-centric solutions to the provision of services (and/or reduction in needs) and a techno-economic comparison with other potential sources of services to meet the power system needs.
The role that wind will play in the solution will be a measure of its competitiveness with respect to other solutions. Resources, policy environment, and the stage of development are different across the world, and there is and will be a wide variety of power systems with distinct characteristics. While most power system needs and services will share a lot in common, some of them will be system specific. Therefore, we recommend that wind technology in this rapidly changing environment adapt competitively, leveraging its advantages and minimising its disadvantages on a regional basis. For example, wind can respond more quickly and more accurately than an SM to meet and provide some services, and these may be an advantage depending on the power system dynamic characteristics of the host power system.
We also recommend that the wind industry now shift towards a more holistic minimisation of the portfolio cost while ensuring higher value of wind power, as well as maintaining and/or improving grid reliability. This requires signals from the regulators, system, and market operators that reward a holistic approach and means that wind power needs to ensure coordination across its own technical capabilities and across multiple timescales to maximise its value to the power system and minimise the cost to the wind technology. Maximising the contribution of wind technology to the power system requires a deep understanding of the behaviour of the wind technology with respect to its capacity to provide services and with respect to how they interact with and/or are dependent on one another. For example, wind power inherently has little stored energy, and as many of the services require energy then the provision of this energy is fundamental and acts to make the services highly dependent on one another. This coordination challenge is dominated by the provision of active power needed during service provision as the active power may also be needed simultaneously for multiple services (frequency control, damping, etc.), which makes the coordination of the services from wind highly complex. For example, the interactions of wake steering, curtailment, and the provision of services have not been fully investigated in detail. Going beyond the turbine and plant, the coordination/aggregation across a region brings challenges that are spatial and temporal. The temporal issue is not only in an operational time frame, as the capital and maintenance cost implications of providing services from wind technology are an important dimension of cost minimisation.
Since the earliest times in the industry, forecasting has been a research challenge. Coordinating services from wind brings a new dimension to the forecasting challenge, and as the energy system becomes more integrated, the coordination and forecasting across wind and solar, demand, new electrified demand, and flexible demands make this an exciting area. Its contribution is manifold, reducing needs, e.g. reduced frequency control, an increase in the reliability of wind service provision, and in the longer term an increase in the reliability of capacity services.
Not all the challenges within wind technology are directly coordination related. For frequency control, some open issues remain for faster responses, like the ability to measure, compute, and transmit the frequency signal to the wind turbine plant controller fast enough. For the best impact on capacity value, diversification of turbine types is the key, i.e. not just focussing on maximising energy capture but accounting for when the wind resource can be converted into electricity by focussing on low wind speeds. This diversification is also evident in offshore wind and possibly in future breakthroughs such as airborne wind and the ability of wind turbines to withstand extreme weather. As the penetration of IBRs increases, there may be an inflection point where, for example, SMs disappear completely from the power system and the need for synchronisation also disappears. However up to that point when SMs are decreasing in number, the need to provide the synchronisation service from IBRs will remain a significant research challenge. These phase-change-type transitions are unknown in detail and may form a significant difference between power systems of the future where there may be several main types, in contrast to today where the synchronous power system is not the only and dominant type. The opportunities for wind and other technologies during these transitions are significant and potentially extremely challenging.
Wind technology interfaces with power systems and with most new devices is dominated by power electronics (IBRs), and many of the potential evolving needs and services are being driven by the characteristics and nature of this enabling technology. Power electronics differ significantly from SMs, principally with respect to its characteristics being determined by the control algorithms deployed within the physical limitations of the hardware, in contrast to SMs where the characteristics are driven almost entirely by the basic electromechanical physics of the hardware. There are positives and negatives in this technology transition best-summarised as positives emanating from the controllability offered by the algorithms but with some physical limitation of the hardware, e.g. over-current capability and the lack of direct mechanical coupling that can provide inertial response.
While the fundamental principles of IBR controls are a research topic regardless of the host technology, the implementation in wind turbines will need to be adjusted or tailored to their characteristics. IBR-based wind power could mimic SMs, but this may not be the best approach as it may negate the controllability advantage. We recommend that the research effort should be focussed on leveraging the unique capabilities of the power electronics that interface wind with the grid. There is a growing realisation that there is no one control approach that is most appropriate, that a mix of control approaches will be best, and that this will be system specific. This is best characterised by the so-called grid-forming or grid-following approaches where, for example, grid-forming mode would significantly decrease the reaction time and, to some extent, mitigate, for example, the challenge of measuring, computing, and transmitting frequency change. But going all in to grid forming is not recommended as it brings its own challenges. Understanding the impacts and benefits of the different control approaches is a power system research focus, but the wind technology needs to be involved and participate so the different control approaches can be designed into the basic wind turbine technologies. We recommend, therefore, that the wind industry work with not only the power systems community but also the power electronics manufacturers to address these research challenges.
Many of the integration challenges can be solved without direct or indirect physical participation of wind technology, and as stated above, this is very system dependent. While wind may not be the central part of a solution, it may play a part, e.g. in protection; while wind may not be capable of providing significant fault current, it can provide some, and this can be part of a bigger solution. Regardless, wind as an integral part of future power systems needs to provide information on its own performance and characteristics so that system operators can, at a system level, determine and quantify the needs. The control algorithms embedded in the power electronics that interface to the power systems cause significant challenges in quantifying the needs. They are vendor specific and proprietary; they expand the degrees of freedom significantly at a device level, and with the relatively small size relative to SM resources, they also expand the spatial dimensionality. This is now causing real problems in many of the leading power systems globally, and therefore robust wind turbine plant models that represent the power electronics and its controls are urgently needed; we recommend that the wind industry work with all stakeholders to bridge this gap. Allied to this challenge is the development of appropriate tools for wind power plants and power system analysis (methods, component models, and data) for both power system planning and operations, and we recommend that the wind industry provide the detailed models and data to empower these tools and methods.
The needs and services paradigm has been employed here to assess research challenges for wind technology with respect to integration challenges. This paradigm is evolving rapidly with new needs and services being identified regularly within the power system operator community (NGESO, 2024) and in academia (Hatziargyriou et al., 2020); therefore, despite the comprehensive version adopted here (Bialek et al., 2021), there are gaps. For example, harmonics are a very important and emerging topic, and wind turbine converters can be tuned to act as an active filter, reducing the harmonic distortion (Kocewiak et al., 2023). Furthermore differences in power systems can result in differences in needs, which in turn may result in fragmentation (IEA, 2023a).
The increasing share of wind power and other technologies is fundamentally altering the nature of power systems. Wind power's role in future power systems will be driven by its ability to adapt to these changes by competitively providing the required power system services. This will require a holistic research and development approach done in coordination with the other technological research communities including solar PV, power electronics, and power systems. A needs and services paradigm is one way of ensuring that the research is targeted at characteristics that are valued by the power system through either market signals or mandates. However, as power systems evolve so will the needs and services – in a way that is not yet fully understood. In this dynamic environment wind research challenges and priorities will need to adapt. Other technologies, in particular solar PV, may also benefit from the recommendations and conclusions contained here. This all needs to be done while recognising the fundamental objective of power systems – maintaining supply–demand balance reliably at the lowest cost.
No data sets were used in this article.
MOM wrote the initial full draft of the paper, proposed the structure and conclusions, edited it extensively throughout the process, and finalised the paper before submission. He did this with the assistance of FRN and AH. FRN was also the main contributor for the extensive referencing, ensuring its accuracy and appropriateness at all stages.
HH and NC, in collaboration with MOM, were the main architects of the structure and content; they also contributed significance to the technical details of many sections and were the main reviewers of the paper with respect to quality control.
TKV, JK, VG, and XW reviewed the paper on several occasions, contributing to fine-tuning several sections related to their own areas of expertise and in general provided feedback on the paper.
At least one of the (co-)authors is a member of the editorial board of Wind Energy Science. The peer-review process was guided by an independent editor, and the authors also have no other competing interests to declare.
Publisher's note: Copernicus Publications remains neutral with regard to jurisdictional claims made in the text, published maps, institutional affiliations, or any other geographical representation in this paper. While Copernicus Publications makes every effort to include appropriate place names, the final responsibility lies with the authors.
This article was written as an international research collaboration under IEA Wind TCP Task 25: “Design and Operation of Energy Systems with Large Amounts of Variable Generation”. It is part of the IEA Wind TCP Grand Challenges set of articles, and the authors would like to acknowledge Paul Veers and Katherine Dykes for guidance. The authors would also like to acknowledge the review comments from Charlie Smith, Damian Flynn, Ana Estanqueiro, Jan Dobschinski, Niina Helistö, Matti Koivisto, Germàn Morales, Deepak Ramasubramanian, Tim Green, and Paul Veers.
This research has been supported by the Leverhulme Trust (grant no. LIP-2020-002).
This paper was edited by Cristina Archer and reviewed by three anonymous referees.
Abdul Wahab, N. I. and Mohamed, A.: Area-based COI-referred rotor angle index for transient stability assessment and control of power systems, Absrt. Appl. Anal., 2012, 410461, https://doi.org/10.1155/2012/410461, 2012.
Achilles, S.: Black Start and System Restoration with Wind and Solar, ESIG – Energy Systems Integration Group, https://www.esig.energy/download/session-a-4-black-start-and-system-restoration-with-wind-and (last access: 11 October 2024), 2018.
Ackermann, T.: Wind power in power systems, 2, John Wiley & Sons, https://doi.org/10.1002/9781119941842.ch4, 2012.
Ackermann, T., Prevost, T., Vittal, V., Roscoe, A. J., Matevosyan, J., and Miller, N.: Paving the way: A future without inertia is closer than you think, IEEE Power Energ. Mag., 15, 61–69, https://doi.org/10.1109/MPE.2017.2729138, 2017.
Ahmed, S. D., Al-Ismail, F. S., Shafiullah, M., Al-Sulaiman, F. A., and El-Amin, I. M.: Grid integration challenges of wind energy: A review, IEEE Access, 8, 10857–10878, https://doi.org/10.1109/ACCESS.2020.2964896, 2020.
Altin, M., Hansen, A. D., Barlas, T. K., Das, K., and Sakamuri, J. N.: Optimization of short-term overproduction response of variable speed wind turbines, IEEE T. Sustain. Energ., 9, 1732–1739, https://doi.org/10.1109/TSTE.2018.2810898, 2018.
Appleby, S. and Rositano, P.: Addressing the System Strength Gap in SA: Economic Evaluation Report, ElectraNet, https://www.aer.gov.au/system/files/ElectraNet-System Strength Economic Evaluation Report- (last access: 11 October 2024), 2019.
Avazov, A., Colas, F., Beerten, J., and Guillaud, X.: Application of input shaping method to vibrations damping in a Type-IV wind turbine interfaced with a grid-forming converter, Elect. Power Syst. Res., 210, 108083, https://doi.org/10.1016/j.epsr.2022.108083, 2022.
Badesa, L., Teng, F., and Strbac, G.: Conditions for regional frequency stability in power system scheduling – Part I: Theory, IEEE T. Power Syst., 36, 5558–5566, https://doi.org/10.1109/TPWRS.2021.3073083, 2021.
Bechtle, P., Schelbergen, M., Schmehl, R., Zillmann, U., and Watson, S.: Airborne wind energy resource analysis, Renew. Energy, 141, 1103–1116, https://doi.org/10.1016/j.renene.2019.03.118, 2019.
Bevrani, H. and Hiyama, T.: Intelligent Automatic Generation Control, CRC Press, ISBN 1439849544, 2011.
Bialek, J., Bowen, T., Green, T., Lew, D., Li, Y., MacDowell, J., Matevosyan, J., Miller, N., O'Malley, M., and Ramasubramanian, D.: System needs and services for systems with high IBR penetration, Global Power System Transformation Consortium, https://globalpst.org/wp-content/uploads/GPST-IBR-Research-Team-System-Services-and-Needs-for (last access: 11 October 2024), 2021.
Bie, Z., Lin, Y., Li, G., and Li, F.: Battling the extreme: A study on the power system resilience, Proc. IEEE, 105, 1253–1266, https://doi.org/10.1109/JPROC.2017.2679040, 2017.
Bird, L., Milligan, M., and Lew, D.: Integrating variable renewable energy: Challenges and solutions, National Renewable Energy Lab, https://doi.org/10.2172/1097911, 2013.
Boldea, I.: Synchronous generators, CRC Press, https://doi.org/10.1201/b19310, 2005.
Bonfiglio, A., Invernizzi, M., Labella, A., and Procopio, R.: Design and implementation of a variable synthetic inertia controller for wind turbine generators, IEEE T. Power Syst., 34, 754–764, https://doi.org/10.1109/TPWRS.2018.2865958, 2018.
Breyer, C., Khalili, S., Bogdanov, D., Ram, M., Oyewo, A. S., Aghahosseini, A., Gulagi, A., Solomon, A., Keiner, D., and Lopez, G.: On the history and future of 100 % renewable energy systems research, IEEE Access, 10, 78176–78218, https://doi.org/10.1109/ACCESS.2022.3193402, 2022.
Camm, E., Behnke, M., Bolado, O., Bollen, M., Bradt, M., Brooks, C., Dilling, W., Edds, M., Hejdak, W., and Houseman, D.: Characteristics of wind turbine generators for wind power plants, in: 2009 IEEE Power & Energy Society General Meeting, 26–30 July 2009, Calgary, AB, Canada, https://doi.org/10.1109/PES.2009.5275330, 2009.
Chaudhuri, B., Ramasubramanian, D., Matevosyan, J., O'Malley, M., Miller, N., Green, T., and Zhou, X.: Rebalancing Needs and Services for Future Grids, IEEE Power Energ. Mag., 22, 30–41, https://doi.org/10.1109/MPE.2023.3342113, 2024.
Chen, L., Du, X., Hu, B., and Blaabjerg, F.: Drivetrain oscillation analysis of grid forming type-IV wind turbine, IEEE T. Energ. Convers., 37, 2321–2337, https://doi.org/10.1109/TEC.2022.3179609, 2022.
Chen, Z., Guerrero, J. M., and Blaabjerg, F.: A review of the state of the art of power electronics for wind turbines, IEEE T. Power Elect., 24, 1859–1875, https://doi.org/10.1109/TPEL.2009.2017082, 2009.
Cheng, Y., Fan, L., Rose, J., Huang, S.-H., Schmall, J., Wang, X., Xie, X., Shair, J., Ramamurthy, J. R., and Modi, N.: Real-world subsynchronous oscillation events in power grids with high penetrations of inverter-based resources, IEEE T. Power Syst., 38, 316–330, https://doi.org/10.1109/TPWRS.2022.3161418, 2022.
Cherubini, A., Papini, A., Vertechy, R., and Fontana, M.: Airborne Wind Energy Systems: A review of the technologies, Renew. Sustain. Energ. Rev., 51, 1461–1476, https://doi.org/10.1016/j.rser.2015.07.053, 2015.
Christensen, P. W.: Grid codes: The Manufacturer's Nightmare, European Wind Energy Association, Warsaw, https://www.ewea.org/ewec2010/fileadmin/ewec2010_files/documents/side_events/Peter_Wibaek_Christensen.pdf (last access: 11 October 2024), 2010.
Cochran, J., Miller, M., Zinaman, O., Milligan, M., Arent, D., Palmintier, B., O'Malley, M., Mueller, S., Lannoye, E., and Tuohy, A.: Flexibility in 21st century power systems, National Renewable Energy Lab, https://www.nrel.gov/docs/fy15osti/63021.pdf (last access: 11 October 2024), 2014.
Cradden, L. C., McDermott, F., Zubiate, L., Sweeney, C., and O'Malley, M.: A 34-year simulation of wind generation potential for Ireland and the impact of large-scale atmospheric pressure patterns, Renew. Energy, 106, 165–176, https://doi.org/10.1016/j.renene.2016.12.079, 2017.
Cutululis, N. A., Blaabjerg, F., Østergaard, J., Bak, C. L., Anderson, M., da Silva, F. M. F., Johannsson, H., Wang, X., and Jørgensen, B. H.: The Energy Islands: A Mars Mission for the Energy system, https://vbn.aau.dk/ws/files/445608701/The_Energy_Islands_a_Mars_mission_for_the_Danish_energy_system.pdf (last access: 11 October 2024), 2021.
Dalla Riva, A., Hethey, J., and Vītiòa, A.: IEA Wind TCP Task 26: Impacts of Wind Turbine Technology on the System Value of Wind in Europe, International Energy Agency, https://doi.org/10.2172/1437346, 2017.
Delille, G., Francois, B., and Malarange, G.: Dynamic frequency control support by energy storage to reduce the impact of wind and solar generation on isolated power system's inertia, IEEE T. Sustain. Energ., 3, 931–939, https://doi.org/10.1109/TSTE.2012.2205025, 2012.
Denholm, P., Mai, T., Kenyon, R. W., Kroposki, B., and O'Malley, M.: Inertia and the power grid: A guide without the spin, National Renewable Energy Lab, https://doi.org/10.2172/1659820, 2020.
Denholm, P., Arent, D. J., Baldwin, S. F., Bilello, D. E., Brinkman, G. L., Cochran, J. M., Cole, W. J., Frew, B., Gevorgian, V., Heeter, J., Hodge, B.-M., Kroposki, B., Mai, T., O'Malley, M., Palmintier, B., Steinberg, D., and Zhang, Y.: The challenges of achieving a 100 % renewable electricity system in the United States, Joule, 5, 1331–1352, https://doi.org/10.1016/j.joule.2021.03.028, 2021.
Denholm, P. L., Sun, Y., and Mai, T. T.: An introduction to grid services: Concepts, technical requirements, and provision from wind, National Renewable Energy Lab, https://doi.org/10.2172/1493402, 2019.
Denis, G., Prevost, T., Debry, M. S., Xavier, F., Guillaud, X., and Menze, A.: The Migrate project: the challenges of operating a transmission grid with only inverter-based generation. A grid-forming control improvement with transient current-limiting control, IET Renew. Power Gen., 12, 523–529, https://doi.org/10.1049/iet-rpg.2017.0369, 2018.
Denny, E. and O'Malley, M.: Quantifying the total net benefits of grid integrated wind, IEEE T. Power Syst., 22, 605–615, https://doi.org/10.1109/TPWRS.2007.894864, 2007.
Diógenes, J. R. F., Claro, J., Rodrigues, J. C., and Loureiro, M. V.: Barriers to onshore wind energy implementation: A systematic review, Energ. Res. Social Sci., 60, 101337, https://doi.org/10.1016/j.erss.2019.101337, 2020.
Doherty, R., Lalor, G., and O'Malley, M.: Frequency control in competitive electricity market dispatch, IEEE T. Power Syst., 20, 1588–1596, https://doi.org/10.1109/TPWRS.2005.852146, 2005.
Domínguez-García, J. L., Gomis-Bellmunt, O., Bianchi, F. D., and Sumper, A.: Power oscillation damping supported by wind power: A review, Renew. Sustain. Energ. Rev., 16, 4994–5006, https://doi.org/10.1016/j.rser.2012.03.063, 2012.
Dykes, K., Kitzing, L., Andersson, M., Pons-Seres de Brauwer, C., and Canét, H.: Beyond LCOE: New assessment criteria for evaluating Wind Energy R&I, in: 2020 Beyond LCOE Workshop, 23–24 January 2020, Brussels, Belgium, SETWind, https://backend.orbit.dtu.dk/ws/portalfiles/portal/234026713/Beyond_LCOE_New_Assessment_Criteria_for_Evaluating_Wind_Energy_RI.pdf (last access: 11 October 2024), 2020.
Dykes, K. L., Veers, P. S., Lantz, E. J., Holttinen, H., Carlson, O., Tuohy, A., Sempreviva, A. M., Clifton, A., Rodrigo, J. S., and Berry, D. S.: IEA wind TCP: Results of IEA wind TCP workshop on a grand vision for wind energy technology, National Renewable Energy Lab, https://doi.org/10.2172/1508509, 2019.
EAWE – European Academy of Wind Energy: Grand Challenges: wind energy research needs for a global energy transition, https://www.wind-energy-science.net/articles_and_preprints/grand-challenges.html (last access: 12 October 2024), 2023.
EIA: Levelized Costs of New Generation Resources in the Annual Energy Outlook 2022, EIA, https://www.eia.gov/outlooks/aeo/pdf/electricity_generation.pdf (last access: 11 October 2024), 2022.
EirGrid: All island grid study overview, EirGrid Group, https://cms.eirgrid.ie/sites/default/files/publications/11-AllIslandGridStudyStudyOverviewJan08.pdf (last access: 11 October 2024), 2008.
EirGrid: EirGrid Grid Code, EirGrid, https://cms.eirgrid.ie/sites/default/files/publications/Grid-Code.pdf (last access: 11 October 2024), 2019.
EirGrid: DS3 Programme, https://www.eirgridgroup.com/how-the-grid-works/ds3-programme/ (last access: 11 October 2024), 2023.
Ela, E., Gevorgian, V., Tuohy, A., Kirby, B., Milligan, M., and O'Malley, M.: Market designs for the primary frequency response ancillary service – Part I: Motivation and design, IEEE T. Power Syst., 29, 421–431, https://doi.org/10.1109/TPWRS.2013.2264942, 2013.
Ela, E., Gevorgian, V., Fleming, P., Zhang, Y., Singh, M., Muljadi, E., Scholbrook, A., Aho, J., Buckspan, A., Pao, L., Shigvi, V., Tuohy, A., Pourbeik, P., Brooks, D., and Bhatt, N.: Active power controls from wind power: Bridging the gaps, NREL, https://doi.org/10.2172/1117060, 2014.
Ela, E., Wang, C., Moorty, S., Ragsdale, K., O'Sullivan, J., Rothleder, M., and Hobbs, B.: Electricity markets and renewables: A survey of potential design changes and their consequences, IEEE Power Energ. Mag., 15, 70–82, https://doi.org/10.1109/MPE.2017.2730827, 2017.
Ela, E., Hytowitz, R., and Helman, U.: Ancillary services in the united states: Technical requirements, market designs, and price trends, EPRI – Electric Power Research Institute, https://www.epri.com/research/products/000000003002015670 (last access: 11 October 2024), 2019.
Ela, E., Mills, A., Gimon, E., Hogan, M., Bouchez, N., Giacomoni, A., Ng, H., Gonzalez, J., and DeSocio, M.: Electricity market of the future: potential North American designs without fuel costs, IEEE Power Energ. Mag., 19, 41–52, https://doi.org/10.1109/MPE.2020.3033396, 2021.
El-Naggar, A. and Erlich, I.: Fault current contribution analysis of doubly fed induction generator-based wind turbines, IEEE T. Energ. Convers., 30, 874–882, https://doi.org/10.1109/TEC.2015.2398671, 2015.
EPRI: Resource Adequacy Philosophy: A Guide to Resource Adequacy Concepts and Approaches, https://www.epri.com/research/programs/067417/results/3002024368 (last access: 11 October 2024), 2022a.
EPRI: Resource Adequacy for a Decarbonized Future A Summary of Existing and Proposed Resource Adequacy Metrics, https://www.epri.com/research/programs/067417/results/3002023230 (last access: 11 October 2024), 2022b.
ESIG: Toward 100 % Renewable Energy Pathway: Key Research Needs, ESIG, https://www.esig.energy/wp-content/uploads/2020/06/Toward-100-Renewable-Energy-Pathways-Key-Research-Needs.pdf (last access: 11 October 2024), 2019.
Eto, J. H., Undrill, J., Mackin, P., Daschmans, R., Williams, B., Haney, B., Hunt, R., Ellis, J., Illian, H., Martinez, C., O'Malley, M., Coughlin, K., and LaCommare, K. H.: Use of frequency response metrics to assess the planning and operating requirements for reliable integration of variable renewable generation, Lawrence Berkeley National Lab, https://doi.org/10.2172/1003830, 2010.
GE: Riders On The Storm: GE Is Building A Wind Turbine That Can Weather Violent Typhoons, Hurricanes, https://w3.windfair.net/wind-energy/pr/28786-ge-ge-renewable-energy-turbine-wind-turbine-typhoon (last access: 11 October 2024), 2018.
Gevorgian, V., Shah, S., Yan, W., and Henderson, G.: Grid-forming wind: getting ready for prime time, with or without inverters, IEEE Electr. Mag., 10, 52–64, https://doi.org/10.1109/MELE.2021.3139246, 2022.
Ghasemi, H., Gharehpetian, G., Nabavi-Niaki, S. A., and Aghaei, J.: Overview of subsynchronous resonance analysis and control in wind turbines, Renew. Sustain. Energ. Rev., 27, 234–243, https://doi.org/10.1016/j.rser.2013.06.025, 2013.
Ghosh, S., Isbeih, Y. J., Bhattarai, R., El Moursi, M. S., El-Saadany, E. F., and Kamalasadan, S.: A dynamic coordination control architecture for reactive power capability enhancement of the DFIG-based wind power generation, IEEE T. Power Syst., 35, 3051–3064, https://doi.org/10.1109/TPWRS.2020.2968483, 2020.
Girsang, I. P., Dhupia, J. S., Singh, M., Gevorgian, V., Muljadi, E., and Jonkman, J.: Impacts of providing inertial response on dynamic loads of wind turbine drivetrains, in: 2014 IEEE Energy Conversion Congress and Exposition (ECCE), 14–18 September 2014, Pittsburgh, PA, USA, https://doi.org/10.1109/ECCE.2014.6953597, 2014.
Glasdam, J. B., Zeni, L., Gryning, M., Hjerrild, J., Kocewiak, Ł., Hesselbaek, B., Andersen, K., Sørensen, T., Blanke, M., and Sørensen, P. E.: HVDC connected offshore wind power plants: review and outlook of current research, in: Event 12th International Workshop on Large-Scale Integration of Wind Power into Power Systems as well as on Transmission Networks for Offshore Wind Power Plants, 22–24 October 2013 London, UK, ISBN 978-3-98-13870-7-0, https://findit.dtu.dk/en/catalog/537f109374bed2fd2100e8e1 (last access: 11 October 2024), 2013.
Gloe, A., Jauch, C., Craciun, B., Zanter, A., and Winkelmann, J.: Influence of continuous provision of synthetic inertia on the mechanical loads of a wind turbine, Energies, 14, 5185, https://doi.org/10.3390/en14165185, 2021.
Glover, J. D., Sarma, M. S., and Overbye, T.: Power system analysis & design, SI version, Cengage Learning, Cengage Learning, ISBN 9780357676387, https://www.cengage.uk/c/power-system-analysis-and-design-si-edition-7e-glover-sarma (last access: 11 October 2024), 2012.
Göçmen, T., Giebel, G., Poulsen, N. K., and Sørensen, P. E.: Possible power of down-regulated offshore wind power plants: The PossPOW algorithm, Wind Energy, 22, 205–218, https://doi.org/10.1002/we.2279, 2018.
Gonzalez-Longatt, F. M., Acosta, M. N., Chamorro, H. R., and Rueda Torres, J. L.: Power converters dominated power systems, in: Modelling and Simulation of Power Electronic Converter Dominated Power Systems in PowerFactory, Springer, 1–35, https://doi.org/10.1007/978-3-030-54124-8_1, 2021.
Government of Ireland: Climate action plan 2021 – Securing our future, Government of Ireland, https://assets.gov.ie/203558/f06a924b-4773-4829-ba59-b0feec978e40.pdf, (last access: 11 October 2024) 2021.
Gu, M., Meegahapola, L., and Wong, K. L.: Coordinated voltage and frequency control in hybrid AC/MT-HVDC power grids for stability improvement, IEEE T. Power Syst., 36, 635–647, https://doi.org/10.1109/TPWRS.2020.2983431, 2020.
Haegel, N. M., Atwater Jr., H., Barnes, T., Breyer, C., Burrell, A., Chiang, Y.-M., De Wolf, S., Dimmler, B., Feldman, D., and Glunz, S.: Terawatt-scale photovoltaics: Transform global energy, Science, 364, 836–838, https://doi.org/10.1126/science.aaw1845, 2019.
Hansen, A. D., Altin, M., Margaris, I. D., Iov, F., and Tarnowski, G. C.: Analysis of the short-term overproduction capability of variable speed wind turbines, Renew. Energy, 68, 326–336, https://doi.org/10.1016/j.renene.2014.02.012, 2014.
Hasche, B., Keane, A., and O'Malley, M.: Capacity value of wind power, calculation, and data requirements: the Irish power system case, IEEE T. Power Syst., 26, 420–430, https://doi.org/10.1109/TPWRS.2010.2051341, 2010.
Hatziargyriou, N., Milanovic, J., Rahmann, C., Ajjarapu, V., Canizares, C., Erlich, I., Hill, D., Hiskens, I., Kamwa, I., and Pal, B.: Definition and classification of power system stability–revisited & extended, IEEE T. Power Syst., 36, 3271–3281, https://doi.org/10.1109/TPWRS.2020.3041774, 2020.
Hedman, K. W., Oren, S. S., and O'Neill, R. P.: A review of transmission switching and network topology optimization, in: 2011 IEEE Power and Energy Society General Meeting, 24–28 July 2011, Detroit, MI, USA, 1–7, https://doi.org/10.1109/PES.2011.6039857, 2011.
Henderson, C.: Interactions of grid-forming converters for windfarm applications, Department of Electronic and Electrical Engineering, University of Strathclyde, https://doi.org/10.48730/0qk4-kk24, 2023.
Henderson, G.: The latest development in synchronous wind turbine technology: how the LVS system can deliver low cost, broad-band variable turbine speed and type 5 grid connection, https://doi.org/10.1049/icp.2021.2636, 2021.
Henderson, G. and Gevorgian, V.: Type 5 wind turbine technology: how synchronised, synchronous generation avoids uncertainties about inverter interoperability under IEEE 2800: 2022, in: 21st Wind & Solar Integration Workshop, Hybrid Conference, 12–14 October 2022, the Hague, the Netherlands, https://doi.org/10.1049/icp.2022.2764, 2022.
Hobbs, B. F., Wang, Y., Xu, Q., Zhang, S., Hamann, H. F., Zhang, R., Siebenschuh, C., Zhang, J., Li, B., and He, L.: Coordinated ramping product and regulation reserve procurements in caiso and miso using multi-scale probabilistic solar power forecasts (pro2r), Johns Hopkins Univ., Baltimore, MD, https://doi.org/10.2172/1873393, 2022.
Hodge, B. M. S., Jain, H., Brancucci, C., Seo, G. S., Korpås, M., Kiviluoma, J., Holttinen, H., Smith, J. C., Orths, A., and Estanqueiro, A.: Addressing technical challenges in 100 % variable inverter-based renewable energy power systems, Wiley Interdisciplin. Rev.: Energ. Environ., 9, e376, https://doi.org/10.1002/wene.376, 2020.
Hogan, W. W.: On an “energy only” electricity market design for resource adequacy, Harvard University, https://www.lmpmarketdesign.com/papers/Hogan_Energy_Only_092305.pdf (last access: 11 October 2024), 2005.
Holttinen, H.: IEA Wind Annual Report 2022, IEA, https://iea-wind.org/wp-content/uploads/2023/10/IEA_Wind_TCP_Annual_Report_2022_ExecutiveSummary.pdf (last access: 11 October 2024), 2023.
Holttinen, H., Kiviluoma, J., Levy, T., Jun, L., Eriksen, P. B., Orths, A., Cutululis, N., Silva, V., Neau, E., and Dobschinski, J.: Design and operation of power systems with large amounts of wind power: Final summary report, IEA WIND Task 25, Phase four 2015-20179513886832, IEA, https://doi.org/10.32040/2242-122X.2019.T350, 2019.
Holttinen, H., Kiviluoma, J., Flynn, D., Smith, J. C., Orths, A., Eriksen, P. B., Cutululis, N., Söder, L., Korpås, M., Estanqueiro, A., MacDowell, J., Tuohy, A., Vrana, T. K., and O'Malley, M.: System impact studies for near 100 % renewable energy systems dominated by inverter based variable generation, IEEE T. Power Syst., 37, 3249–3258, https://doi.org/10.1109/TPWRS.2020.3034924, 2020.
Holttinen, H., Kiviluoma, J., Helistö, N., Levy, T., Menemenlis, N., Jun, L., Cutululis, N. A., Koivisto, M., Das, K., and Orths, A.: Design and operation of energy systems with large amounts of variable generation: Final summary report, IEA Wind TCP Task 25, VTT Technical Research Centre of Finland, 951388757X, https://doi.org/10.32040/2242-122X.2021.T396, 2021.
Houck, D. R.: Review of wake management techniques for wind turbines, Wind Energy, 25, 195–220, https://doi.org/10.1002/we.2668, 2022.
IEA: World Energy Outlook 2022, IEA, https://www.iea.org/reports/world-energy-outlook-2022 (last access: 11 October 2024), 2022.
IEA: Electricity Grids and Secure Energy Transitions, International Energy Agency, https://www.iea.org/reports/electricity-grids-and-secure-energy-transitions (last access: 11 October 2024), 2023a.
IEA: Wind TCP Task 50: https://iea-wind.org/task50/ (last access: 11 October 2024), 2023b.
IRENA: Grid Codes for Renewable Powered Systems, Abu Dhabi, https://www.irena.org/-/media/Files/IRENA/Agency/Publication/2022/Apr/IRENA_Grid_Codes_Renewable_Systems_2022.pdf?rev=986f108cbe5e47b98d17fca93eee6c86 (last access: 11 October 2024), 2022.
Jain, A., Saborío-Romano, O., Sakamuri, J. N., and Cutululis, N. A.: Blackstart from HVDC-connected offshore wind: Hard versus soft energization, IET Renew. Power Gen., 15, 127–138, https://doi.org/10.1049/rpg2.12010, 2020.
Jorgenson, J., Denholm, P., and Mai, T.: Analyzing storage for wind integration in a transmission-constrained power system, Appl. Energ., 228, 122–129, https://doi.org/10.1016/j.apenergy.2018.06.046, 2018.
Keane, A., Milligan, M., Dent, C. J., Hasche, B., D'Annunzio, C., Dragoon, K., Holttinen, H., Samaan, N., Soder, L., and O'Malley, M.: Capacity value of wind power, IEEE T. Power Syst., 26, 564–572, https://doi.org/10.1109/TPWRS.2010.2062543, 2011.
Kemp, J. M., Millstein, D., Kim, J. H., and Wiser, R.: Interactions between hybrid power plant development and local transmission in congested regions, Adv. Appl. Energ., 10, 100133, https://doi.org/10.1016/j.adapen.2023.100133, 2023.
Kirkegaard, J. K., Rudolph, D. P., Nyborg, S., Solman, H., Gill, E., Cronin, T., and Hallisey, M.: Tackling grand challenges in wind energy through a socio-technical perspective, Nat. Energ., 8, 655–664, https://doi.org/10.1038/s41560-023-01266-z, 2023.
Kirschen, D. S. and Strbac, G.: Fundamentals of power system economics, John Wiley & Sons, ISBN 111921324X, 2018.
Kocewiak, L., Guest, E., Dowlatabadi, M. K. B., Chen, S., Árnadóttir, U. D., Jensen, S. J., Kramer, B. Ø., Nagaiceva, V., Siepker, T., and Terriche, Y.: Active filtering trial to reduce harmonic voltage distortion in an offshore wind power plant, in: 22nd Wind and Solar Integration Workshop, 26–28 September 2023, Copenhagen, Denmark, 394–399, https://doi.org/10.1049/icp.2023.2764, 2023.
Kolar, J. W., Friedli, T., Krismer, F., Looser, A., Schweizer, M., Friedemann, R. A., Steimer, P. K., and Bevirt, J. B.: Conceptualization and multiobjective optimization of the electric system of an airborne wind turbine, IEEE J. Emerg. Sel. Top. Power Elect., 1, 73–103, https://doi.org/10.1109/JESTPE.2013.2269672, 2013.
Kroposki, B., Johnson, B., Zhang, Y., Gevorgian, V., Denholm, P., Hodge, B.-M., and Hannegan, B.: Achieving a 100 % renewable grid: Operating electric power systems with extremely high levels of variable renewable energy, IEEE Power Energ. Mag., 15, 61–73, https://doi.org/10.1109/MPE.2016.2637122, 2017.
Lannoye, E., Flynn, D., and O'Malley, M.: Evaluation of power system flexibility, IEEE T. Power Syst., 27, 922–931, https://doi.org/10.1109/TPWRS.2011.2177280, 2012.
Lauby, M. G., Ahlstrom, M., Brooks, D. L., Beuning, S., Caspary, J., Grant, W., Kirby, B., Milligan, M., O'Malley, M., Patel, M., Piwko, R., Pourbeik, P., Shirmohammadi, D., and Smith, C. J.: Balancing Act, IEEE Power Energ. Mag., 9, 75–85, https://doi.org/10.1109/MPE.2011.942352, 2011.
Lazard: Lazard's Levelized Cost of Energy Analysis, https://www.lazard.com/media/2ozoovyg/lazards-lcoeplus-april-2023.pdf (last access: 11 October 2024), 2023.
Leutbecher, M. and Palmer, T. N.: Ensemble forecasting, J. Comput. Phys., 227, 3515–3539, https://doi.org/10.1016/j.jcp.2007.02.014, 2008.
Lin, Y., Eto, J. H., Johnson, B. B., Flicker, J. D., Lasseter, R. H., Villegas Pico, H. N., Seo, G.-S., Pierre, B. J., and Ellis, A.: Research roadmap on grid-forming inverters, NREL – National Renewable Energy Lab., Golden, CO, USA, https://doi.org/10.2172/1721727, 2020.
Lin, Y., Eto, J. H., Johnson, B. B., Flicker, J. D., Lasseter, R. H., Pico, H. N. V., Seo, G.-S., Pierre, B. J., Ellis, A., Miller, J., and Yuan, G.: Pathways to the next-generation power system with inverter-based resources: Challenges and recommendations, IEEE Power Energ. Mag., 10, 10–21, https://doi.org/10.1109/MELE.2021.3139132, 2022.
Lin, Z. and Liu, X.: Wind power forecasting of an offshore wind turbine based on high-frequency SCADA data and deep learning neural network, Energy, 201, 117693, https://doi.org/10.1016/j.energy.2020.117693, 2020.
Liu, J., Yao, Q., and Hu, Y.: Model predictive control for load frequency of hybrid power system with wind power and thermal power, Energy, 172, 555–565, https://doi.org/10.1016/j.energy.2019.01.071, 2019.
Liu, T. and Wang, X.: Transient stability of single-loop voltage-magnitude controlled grid-forming converters, IEEE T. Power Elect., 36, 6158–6162, https://doi.org/10.1109/TPEL.2020.3034288, 2021.
Loth, E., Qin, C., Simpson, J. G., and Dykes, K.: Why we must move beyond LCOE for renewable energy design, Adv. Appl. Energ., 8, 100112, https://doi.org/10.1016/j.adapen.2022.100112, 2022.
Lu, L., Saborío-Romano, O., and Cutululis, N. A.: Torsional oscillation damping in wind turbines with virtual synchronous machine-based frequency response, Wind Energy, 25, 1157–1172, https://doi.org/10.1002/we.2719, 2022.
Mahzarnia, M., Moghaddam, M. P., Baboli, P. T., and Siano, P.: A Review of the Measures to Enhance Power Systems Resilience, IEEE Syst. J., 14, 4059–4070, https://doi.org/10.1109/JSYST.2020.2965993, 2020.
Mallapragada, D. S., Sepulveda, N. A., and Jenkins, J. D.: Long-run system value of battery energy storage in future grids with increasing wind and solar generation, Appl. Energ., 275, 115390, https://doi.org/10.1016/j.apenergy.2020.115390, 2020.
Martínez-Turégano, J., Añó-Villalba, S., Bernal-Perez, S., Peña, R., and Blasco-Gimenez, R.: Small-signal stability and fault performance of mixed grid forming and grid following offshore wind power plants connected to a HVDC-diode rectifier, IET Renew. Power Gen., 14, 2166–2175, https://doi.org/10.1049/iet-rpg.2019.1264, 2020.
Matevosyan, J., Badrzadeh, B., Prevost, T., Quitmann, E., Ramasubramanian, D., Urdal, H., Achilles, S., MacDowell, J., Huang, S. H., Vital, V., O'Sullivan, J., and Quint, R.: Grid-forming inverters: Are they the key for high renewable penetration?, IEEE Power Energ. Mag., 17, 89–98, https://doi.org/10.1109/MPE.2019.2933072, 2019.
Matevosyan, J., MacDowell, J., Miller, N., Badrzadeh, B., Ramasubramanian, D., Isaacs, A., Quint, R., Quitmann, E., Pfeiffer, R., Urdal, H., Prevost, T., Vittal, V., Woodford, D., Huang, S. H., and O'Sullivan, J.: A future with inverter-based resources: Finding strength from traditional weakness, IEEE Power Energ. Mag., 19, 18–28, https://doi.org/10.1109/MPE.2021.3104075, 2021.
Meyers, J., Bottasso, C., Dykes, K., Fleming, P., Gebraad, P., Giebel, G., Göçmen, T., and Van Wingerden, J.-W.: Wind farm flow control: prospects and challenges, Wind Energ. Sci., 7, 2271–2306, https://doi.org/10.5194/wes-7-2271-2022, 2022.
Miller, N., Green, T., Li, Y., Ramasubramanian, D., Bialek, J., O'Malley, M., Smith, C., Lew, D., Matevosyan, J., Taul, M. G., and Philbrick, R.: Stability Tools Inventory: Status and Needs, Global Power System Transformation Consortium, 23 August 2021, https://globalpst.org/wp-content/uploads/Tools-Team-Presentation.pdf (last access: 11 October 2024), 2021.
Moawwad, A., El Moursi, M. S., and Xiao, W.: A novel transient control strategy for VSC-HVDC connecting offshore wind power plant, IEEE T. Sustain. Energ., 5, 1056–1069, https://doi.org/10.1109/TSTE.2014.2325951, 2014.
Mohseni, M. and Islam, S. M.: Review of international grid codes for wind power integration: Diversity, technology and a case for global standard, Renew. Sustain. Energ. Rev., 16, 3876–3890, https://doi.org/10.1016/j.rser.2012.03.039, 2012.
Morales-España, G., Nycander, E., and Sijm, J.: Reducing CO2 emissions by curtailing renewables: Examples from optimal power system operation, Energ. Econ., 99, 105277, https://doi.org/10.1016/j.eneco.2021.105277, 2021.
Muljadi, E., Gevorgian, V., Singh, M., and Santoso, S.: Understanding inertial and frequency response of wind power plants, in: IEEE Symposium on Power Electronics and Machines in Wind Applications, 16–18 July 2012, Denver, Colorado, https://doi.org/10.1109/PEMWA.2012.6316361, 2012.
Mullane, A. and O'Malley, M.: The inertial response of induction-machine-based wind turbines, IEEE T. Power Syst., 20, 1496–1503, https://doi.org/10.1109/TPWRS.2005.852081, 2005.
Mullane, A. and O'Malley, M.: Modifying the inertial response of power-converter based wind turbine generators, in: 3rd IET International Conference on Power Electronics, Machines and Drives (PEMD 2006), 4–6 April 2006, Dublin, Ireland, https://doi.org/10.1049/cp:20060084, 2006.
Müller, S., Holttinen, H., Taibi, E., Smith, J., Fraile, D., and Vrana, T. K.: System integration costs—A useful concept that is complicated to quantify, in: Proc. 17th Int. Workshop Large-Scale Integr. Wind Power Power Syst. Well Transmiss. Netw. Offshore Wind Power Plants, https://www.researchgate.net/profile/Hannele-Holttinen/publication/333617914_System_Integration_Costs_-_a_Useful_Concept_that_is_Complicated (last access: 11 October 2024), 2018.
Murphy, C. A., Schleifer, A., and Eurek, K.: A taxonomy of systems that combine utility-scale renewable energy and energy storage technologies, Renew. Sustain. Energ. Rev., 139, 110711, https://doi.org/10.1016/j.rser.2021.110711, 2021.
National Academies of Sciences, Engineering, and Medicine: The Future of Electric Power in the United States, National Academies Press, Washington, D.C., 330 pp., https://doi.org/10.17226/25968, 2021.
Nema, P., Nema, R., and Rangnekar, S.: A current and future state of art development of hybrid energy system using wind and PV-solar: A review, Renew. Sustain. Energ. Rev., 13, 2096–2103, https://doi.org/10.1016/j.rser.2008.10.006, 2009.
Neuhoff, K., Richstein, J. C., and Kröger, M.: Reacting to changing paradigms: How and why to reform electricity markets, Energy Policy, 180, 113691, https://doi.org/10.1016/j.enpol.2023.113691, 2023.
NGESO: Black Start from Non-Traditional Generation Technologies, https://etipwind.eu/files/file/agendas/231205-ETIPWind-SRIA.pdf (last access: 11 October 2024), 2019.
NGESO: Electricity System Operator Markets Roadmap, National Grid Electricity System Operator, 2023.
NGESO: Electricity System Operator Markets Roadmap, National Grid Electricity System Operator, https://www.nationalgrideso.com/document/304131/download (last access: 11 October 2024), 2024.
Nguyen, T.-T., Vu, T., Paudyal, S., Blaabjerg, F., and Vu, T. L.: Grid-Forming Inverter-based Wind Turbine Generators: Comprehensive Review, Comparative Analysis, and Recommendations, arXiv [preprint], https://doi.org/10.48550/arXiv.2203.02105, 2022.
Novacheck, J., Sharp, J., Schwarz, M., Donohoo-Vallett, P., Tzavelis, Z., Buster, G., and Rossol, M.: The evolving role of extreme weather events in the US power system with high levels of variable renewable energy, NREL – National Renewable Energy Lab., Golden, CO, USA, https://doi.org/10.2172/1837959, 2021.
O'Malley, M.: Grid integration [in my view], IEEE Power Energ. Mag., 9, 118–120, https://doi.org/10.1109/MPE.2011.942477, 2011.
O'Malley, M.: Towards 100 % renewable energy system, IEEE T. Power Syst., 37, 3187–3189, https://doi.org/10.1109/TPWRS.2022.3178170, 2022.
O'Malley, M., Kroposki, B., Hannegan, B., Madsen, H., Andersson, M., D'haeseleer, W., McGranaghan, M. F., Dent, C., Strbac, G., Baskaran, S., and Rinker, M.: Energy Systems Integration. Defining and Describing the Value Proposition, United States, Medium: ED; p. 12, OSTI.GOV, https://doi.org/10.2172/1257674, 2016.
Osman, M., Segal, N., Najafzadeh, A., and Harris, J.: Short-circuit modeling and system strength, North American Electric Reliability Corporation, https://www.nerc.com/pa/RAPA/ra/Reliability Assessments DL/Short_Circuit_whitepaper_Final_1_26_18.pdf (last access: 11 October 2024), 2018.
Pagnani, D., Blaabjerg, F., Bak, C. L., Faria da Silva, F. M., Kocewiak, Ł. H., and Hjerrild, J.: Offshore wind farm black start service integration: Review and outlook of ongoing research, Energies, 13, 6286, https://doi.org/10.3390/en13236286, 2020.
Pan, D., Wang, X., Liu, F., and Shi, R.: Transient stability of voltage-source converters with grid-forming control: A design-oriented study, IEEE J. Emerg. Select. Top. Power Elect., 8, 1019–1033, https://doi.org/10.1109/JESTPE.2019.2946310, 2020.
Panteli, M. and Mancarella, P.: The grid: Stronger, bigger, smarter: Presenting a conceptual framework of power system resilience, IEEE Power Energ. Mag., 13, 58–66, https://doi.org/10.1109/MPE.2015.2397334, 2015.
Papadopoulos, P. N. and Milanović, J. V.: Probabilistic framework for transient stability assessment of power systems with high penetration of renewable generation, IEEE T. Power Syst., 32, 3078–3088, https://doi.org/10.1109/TPWRS.2016.2630799, 2016.
Pineda, I. and Vannoorenberghe, C.: Strategic Research & Innovation Agenda 2025–2027, European Technology and Innovation Platform on Wind energy, https://etipwind.eu/files/file/agendas/231205-ETIPWind-SRIA.pdf (last access: 11 October 2024), 2023.
Qiao, W., Harley, R. G., and Venayagamoorthy, G. K.: Coordinated reactive power control of a large wind farm and a STATCOM using heuristic dynamic programming, IEEE T. Energ. Convers., 24, 493–503, https://doi.org/10.1109/TEC.2008.2001456, 2009.
Rafique, Z., Khalid, H. M., Muyeen, S., and Kamwa, I.: Bibliographic review on power system oscillations damping: An era of conventional grids and renewable energy integration, Int. J. Elect. Power Energ. Syst., 136, 107556, https://doi.org/10.1016/j.ijepes.2021.107556, 2022.
Rebours, Y. G., Kirschen, D. S., Trotignon, M., and Rossignol, S.: A survey of frequency and voltage control ancillary services – Part II: Economic features, IEEE T. Power Syst., 22, 358–366, https://doi.org/10.1109/TPWRS.2006.888965, 2007a.
Rebours, Y. G., Kirschen, D. S., Trotignon, M., and Rossignol, S.: A survey of frequency and voltage control ancillary services – Part I: Technical features, IEEE T. Power Syst., 22, 350–357, https://doi.org/10.1109/TPWRS.2006.888963, 2007b.
REN21: Renewables 2023 Global Status Report, https://doi.org/10.1109/TPWRS.2006.888963, 2023.
Roscoe, A., Brogan, P., Elliott, D., Knueppel, T., Gutierrez, I., Crolla, P., Silva, R., Campion, J.-C. P., and Da Silva, R.: Practical experience of providing enhanced grid forming services from an onshore wind park, in: 18th Wind Integration Workshop, 16–18 October 2019, Dublin, Ireland, https://knowledge.rtds.com/hc/en-us/article_attachments/1500001877941/Practical_Experience_of_Operating_a_Grid_Forming_Wind_Park_and_its_Response_to_System_Events.pdf (last access: 11 October 2024), 2020.
Roscoe, A., Knueppel, T., Da Silva, R., Brogan, P., Gutierrez, I., Elliott, D., and Perez Campion, J. C.: Response of a grid forming wind farm to system events, and the impact of external and internal damping, IET Renew. Power Gen., 14, 3908–3917, https://doi.org/10.1049/iet-rpg.2020.0638, 2021.
Sakamuri, J. N., Altin, M., Hansen, A. D., and Cutululis, N. A.: Coordinated frequency control from offshore wind power plants connected to multi terminal DC system considering wind speed variation, IET Renew. Power Gen., 11, 1226–1236, https://doi.org/10.1049/iet-rpg.2016.0433, 2017.
Schuitema, G., Steg, L., and O'Malley, M.: Consumer behavior: why engineers need to read about it [guest editorial], IEEE Power Energ. Mag., 16, 14–48, https://doi.org/10.1109/MPE.2017.2762378, 2018.
Schweppe, F. C., Caramanis, M. C., Tabors, R. D., and Bohn, R. E.: Spot pricing of electricity, Springer Science & Business Media, ISBN 1461316839, 2013.
ScottishPower: Black-Start Capability – A Global first for ScottishPower Renewables, https://www.scottishpowerrenewables.com/pages/innovation.aspx (last access: 11 October 2024), 2023.
SEAI: Wind Energy, https://www.seai.ie/technologies/wind-energy/#:~=Government Supports&text=To achieve this target set,to the grid by 2030 (last access: 11 October 2024), 2023.
Shah, S. and Gevorgian, V.: Control, operation, and stability characteristics of grid-forming type III wind turbines, in: 9th Wind Integration Workshop, 11–12 November 2020, USA, https://www.nrel.gov/docs/fy21osti/78158.pdf (last access: 11 October 2024), 2020.
Simpkins, K.: Inspired by palm trees, scientists develop hurricane-resilient wind turbines, https://www.colorado.edu/today/2022/06/15/inspired-palm-trees-scientists-develop-hurricane-resilient-wind (last access: 11 October 2024), 2022.
Singh, M. and Santoso, S.: Dynamic models for wind turbines and wind power plants, NREL, https://www.nrel.gov/docs/fy12osti/52780.pdf (last access: 11 October 2024), 2011.
Singlitico, A., Campion, N. J. B., Münster, M., Koivisto, M. J., Cutululis, N. A., Suo, C. J., Karlsson, K., Jørgensen, T., Waagstein, J. E., and Bendtsen, M. F.: Optimal placement of P2X facility in conjunction with Bornholm energy island: Preliminary overview for an immediate decarbonisation of maritime transport, Technical University of Denmark, https://orbit.dtu.dk/en/publications/optimal-placement-of-p2x-facility-in-conjunction-with (last access: 11 October 2024), 2020.
Smith, J. C., Milligan, M. R., DeMeo, E. A., and Parsons, B.: Utility wind integration and operating impact state of the art, IEEE T. Power Syst., 22, 900–908, https://doi.org/10.1109/TPWRS.2007.901598, 2007.
Söder, L., Tómasson, E., Estanqueiro, A., Flynn, D., Hodge, B.-M., Kiviluoma, J., Korpås, M., Neau, E., Couto, A., Pudjianto, D., Strbac, G., Burke, D., Gomez, T., Das, K., Cutululis, N., Van Herterm, D., Hoschle, H., Matevosyan, J., Von Roon, S., Carlini, E. M., Gaprabianca, M., and de Vries, L.: Review of wind generation within adequacy calculations and capacity markets for different power systems, Renew. Sustain. Energ. Rev., 119, 109540, https://doi.org/10.1016/j.rser.2019.109540, 2020.
Steg, L., Shwom, R., and Dietz, T.: What drives energy consumers: Engaging people in a sustainable energy transition, IEEE Power Energ. Mag., 16, 20–28, https://doi.org/10.1109/MPE.2017.2762379, 2018.
Stenclik, D., Bloom, A., Cole, W., Figueroa Acevedo, A., Stephen, G., and Touhy, A.: Redefining resource adequacy for modern power systems: A report of the redefining resource adequacy task force, NREL – National Renewable Energy Lab., Golden, CO, USA, https://doi.org/10.2172/1961567, 2021.
Stenclik, D., Goggin, M., Ela, E., and Ahlstrom, M.: Unlocking the Flexibility of Hybrid Resources, Energy Systems Integration Group, https://www.esig.energy/wp-content/uploads/2022/03/ESIG-Hybrid-Resources-report-2022.pdf (last access: 11 October 2024), 2022.
Susskind, L., Chun, J., Gant, A., Hodgkins, C., Cohen, J., and Lohmar, S.: Sources of opposition to renewable energy projects in the United States, Energ Policy, 165, 112922, https://doi.org/10.1016/j.enpol.2022.112922, 2022.
Swisher, P., Leon, J. P. M., Gea-Bermúdez, J., Koivisto, M., Madsen, H. A., and Münster, M.: Competitiveness of a low specific power, low cut-out wind speed wind turbine in North and Central Europe towards 2050, Appl. Energ., 306, 118043, https://doi.org/10.1016/j.apenergy.2021.118043, 2022.
Tande, J. O., Wagenaar, J. W., Latour, M. I., Aubrun, S., Wingerde, A. v., Eecen, P., Andersson, M., Barth, S., McKeever, P., and Cutululis, A. N.: Proposal for European lighthouse project: Floating wind energy, European Energy Research Alliance Wind Energy, https://blogg.sintef.no/wp-content/uploads/2022/03/Lighthouse_SetWind_I.pdf (last access: 11 October 2024), 2022.
Ueckerdt, F., Hirth, L., Luderer, G., and Edenhofer, O.: System LCOE: What are the costs of variable renewables?, Energy, 63, 61–75, https://doi.org/10.1016/j.energy.2013.10.072, 2013.
Van Cutsem, T. and Vournas, C.: Voltage stability of electric power systems, Springer Science & Business Media, https://doi.org/10.1007/978-0-387-75536-6, 2007.
Van Dijk, M. T., Van Wingerden, J.-W., Ashuri, T., and Li, Y.: Wind farm multi-objective wake redirection for optimizing power production and loads, Energy, 121, 561–569, https://doi.org/10.1016/j.energy.2017.01.051, 2017.
Van Nuffel, L., Dedecca, J. G., Smit, T., and Rademaekers, K.: Sector coupling: how can it be enhanced in the EU to foster grid stability and decarbonise?, European Parliament Brussels, Belgium, https://www.europarl.europa.eu/RegData/etudes/STUD/2018/626091/IPOL_STU(2018)626091_EN.pdf (last access: 11 October 2024), 2018.
Veers, P., Dykes, K., Lantz, E., Barth, S., Bottasso, C. L., Carlson, O., Clifton, A., Green, J., Green, P., Holttinen, H., Laird, D., Lehtomaki, V., Lundquist, J. K., Manwell, J., Marquis, M., Meneveau, C., Moriarty, P., Munduate, X., Muskulus, M., Naughton, J., Pao, L., Paquette, J., Peinke, J., Robertson, A., Rodrigo, J. S., Sempreviva, A. M., Smith, C. J., Touhy, A., and Wiser, R.: Grand challenges in the science of wind energy, Science, 366, 6464, https://doi.org/10.1126/science.aau2027, 2019.
Veers, P., Dykes, K., Basu, S., Bianchini, A., Clifton, A., Green, P., Holttinen, H., Kitzing, L., Kosovic, B., Lundquist, J. K., Meyers, J., O'Malley, M., Shaw, W. J., and Straw, B.: Grand Challenges: wind energy research needs for a global energy transition, Wind Energy Sci., 7, 2491–2496, https://doi.org/10.5194/wes-7-2491-2022, 2022.
Veers, P., Bottasso, C. L., Manuel, L., Naughton, J., Pao, L., Paquette, J., Robertson, A., Robinson, M., Ananthan, S., Barlas, T., Bianchini, A., Bredmose, H., Horcas, S. G., Keller, J., Madsen, H. A., Manwell, J., Moriarty, P., Nolet, S., and Rinker, J.: Grand challenges in the design, manufacture, and operation of future wind turbine systems, Wind Energ. Sci., 8, 1071–1131, https://doi.org/10.5194/wes-8-1071-2023, 2023.
Vittal, E., O'Malley, M., and Keane, A.: Rotor angle stability with high penetrations of wind generation, IEEE T. Power Syst., 27, 353–362, https://doi.org/10.1109/TPWRS.2011.2161097, 2011.
Wang, X. and Blaabjerg, F.: Harmonic stability in power electronic-based power systems: Concept, modeling, and analysis, IEEE T. Smart Grid, 10, 2858–2870, https://doi.org/10.1109/TSG.2018.2812712, 2018.
Wilches-Bernal, F., Bidram, A., Reno, M. J., Hernandez-Alvidrez, J., Barba, P., Reimer, B., Montoya, R., Carr, C., and Lavrova, O.: A survey of traveling wave protection schemes in electric power systems, IEEE Access, 9, 72949–72969, https://doi.org/10.1109/ACCESS.2021.3080234, 2021.
WindEurope: Wind Turbine Orders Monitoring Q3 2023, https://windeurope.org/intelligence-platform/reports/ (last access: 11 October 2024), 2023.
Wiser, R., Millstein, D., Bolinger, M., Jeong, S., and Mills, A.: Wind Power Market-Value Enhancements through Larger Rotors and Taller Towers, Energy Systems Integration Group, https://www.esig.energy/download/wind-power-market-value-enhancements-through-larger-rotors (last access: 11 October 2024), 2020.
Wu, Z., Gao, W., Gao, T., Yan, W., Zhang, H., Yan, S., and Wang, X.: State-of-the-art review on frequency response of wind power plants in power systems, J. Mod. Power Syst. Clean Energ., 6, 1–16, https://doi.org/10.1007/s40565-017-0315-y, 2018.
Xu, Y., Zhao, S., Cao, Y., and Sun, K.: Understanding subsynchronous oscillations in DFIG-based wind farms without series compensation, IEEE Access, 7, 107201–107210, https://doi.org/10.1109/ACCESS.2019.2933156, 2019.
Yang, Y., DeFrain, J., and Faruqui, A.: Conceptual discussion on a potential hidden cross-seasonal storage: Cross-seasonal load shift in industrial sectors, Elect. J., 33, 106846, https://doi.org/10.1016/j.tej.2020.106846, 2020.
Zavadil, R., Miller, N., Ellis, A., Muljadi, E., Pourbeik, P., Saylors, S., Nelson, R., Irwin, G., Sahni, M. S., and Muthumuni, D.: Models for change, IEEE Power Energ. Mag., 9, 86–96, https://doi.org/10.1109/MPE.2011.942388, 2011.
Zeni, L.: Power system integration of VSC-HVDC connected offshore wind power plants, DTU Wind Energy, https://orbit.dtu.dk/en/publications/power-system-integration-of-vsc-hvdc-connected-offshore-wind (last access: 11 October 2024), 2015.
Zhang, H., Xiang, W., Lin, W., and Wen, J.: Grid forming converters in renewable energy sources dominated power grid: Control strategy, stability, application, and challenges, J. Mod. Power Syst. Clean Energ., 9, 1239–1256, https://doi.org/10.35833/MPCE.2021.000257, 2021.
Zhao, F., Wang, X., Zhou, Z., Meng, L., Hasler, J.-P., Svensson, J. R., Kocewiak, L., Bai, H., and Zhang, H.: Energy-Storage Enhanced STATCOMs for Wind Power Plants, IEEE Power Elect. Mag., 10, 34–39, https://doi.org/10.1109/MPEL.2023.3273893, 2023.
Zhou, F., Joos, G., and Abbey, C.: Voltage stability in weak connection wind farms, in: IEEE Power Engineering Society General Meeting, 16 June 2005, San Francisco, CA, USA, https://doi.org/10.1109/PES.2005.1489210, 2005.
Zhou, S. and Solomon, B. D.: Do renewable portfolio standards in the United States stunt renewable electricity development beyond mandatory targets?, Energ Policy, 140, 111377, https://doi.org/10.1016/j.enpol.2020.111377, 2020.
While this paper is focused on wind, solar PV has very similar characteristics and impacts on power systems, and therefore they are dealt with together where appropriate, so the solar PV community can also benefit. Wind and solar PV are sometimes collectively referred to as variable renewable energy (VRE) resources, but this collective term is only used in the paper where appropriate, as the focus is on wind.
When the challenge is related to a specific technology sometimes the specific technology is specified, i.e. wind integration. Although wind is the focus here, we will not use the term as one of the key messages is that wind or any technology cannot be treated in isolation from an integration perspective because there are so many changes happening simultaneously.
There is a plethora of terminology that can be confusing, including grid, power grid, network, power system, electricity system, etc. Without defining them strictly, the grid, power grid, and network are all similar and refer to the network of transmission and distribution lines and associated equipment, i.e. the “wires”. The power system and electricity system are similar, power system being the more colloquial term in the engineering community, and including in addition to the grid, the generation, demand, etc. Therefore, the grid and wind are parts of the power system. However, grid has been adopted as the term of common use even when power system may strictly be more correct. Here both grid and power system are used throughout with best endeavours to avoid ambiguity.
Synchronous power systems are dominated by synchronous machines (mainly generators) whose mechanical speeds of rotation are all synchronised together as if they were all mechanically coupled, but this coupling is achieved electrically. Virtually all power systems are synchronous, but this term is not necessarily defined by a geographical, political, and/or commercial boundary but by a technical boundary. Denmark's power system is part of two synchronous power systems: one in the west (part of the much larger European Continental synchronous area) and one in the east (part of the larger Nordic synchronous area). Ireland and Great Britain have their own synchronous power system.
Most SMs (in capacity terms) in power systems are synchronous generators, but other SMs include synchronous condensers and synchronous motors that have very similar characteristics from a services perspective.
Converters go from alternating current (AC) to direct current (DC), and inverters go from DC to AC. For technical reasons, modern wind generation produces AC, and some or all of this is then converted to DC and is then inverted to AC that is injected into the AC grid. Inverter and converter are sometimes used interchangeably.
The simple definition of non-synchronous is that they are not synchronous machines and do not have the inherent physical characteristic of coupling (synchronising) their mechanical rotation; i.e. the power electronics decouples the mechanical inertia of the wind turbine from the grid. However, in a synchronous power system, the electrical frequency of voltages and currents must be coupled to the electrical frequency of the power system (50 or 60 Hz), and this is achieved by the power electronic controls.
These primary energy sources can also be variable due to, e.g. gas supply limitations or drought. This broadening-out of what can impact resource adequacy is part of the evolution of the methodologies.
Not to be confused with capacity value, defined above. The capacity factor is a metric that is more related to minimising the LCOE, whereas the capacity value is directed towards maximising value.
- Abstract
- Introduction
- Integration of wind power in power systems
- Energy and capacity
- Frequency and voltage control
- Synchronisation and damping
- Protection and restoration
- Discussion and recommendations
- Conclusions
- Data availability
- Author contributions
- Competing interests
- Disclaimer
- Acknowledgements
- Financial support
- Review statement
- References
- Abstract
- Introduction
- Integration of wind power in power systems
- Energy and capacity
- Frequency and voltage control
- Synchronisation and damping
- Protection and restoration
- Discussion and recommendations
- Conclusions
- Data availability
- Author contributions
- Competing interests
- Disclaimer
- Acknowledgements
- Financial support
- Review statement
- References