the Creative Commons Attribution 4.0 License.
the Creative Commons Attribution 4.0 License.
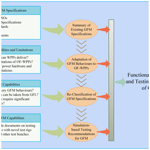
Functional specifications and testing requirements for grid-forming offshore wind power plants
Sulav Ghimire
Gabriel Miguel Gomes Guerreiro
Kanakesh Vatta Kkuni
Emerson David Guest
Kim Høj Jensen
Guangya Yang
Xiongfei Wang
Throughout the past few years, various transmission system operators (TSOs) and research institutes have defined several functional specifications for grid-forming (GFM) converters via grid codes, white papers, and technical documents. These institutes and organisations also proposed testing requirements for general inverter-based resources (IBRs) and specific GFM converters. This paper initially reviews functional specifications and testing requirements from several sources to create an understanding of GFM capabilities in general. Furthermore, it proposes an outlook on the defined GFM capabilities, functional specifications, and testing requirements for offshore wind power plant (OF WPP) applications from an original equipment manufacturer (OEM) perspective. Finally, this paper briefly establishes the relevance of new testing methodologies for equipment-level certification and model validation, focusing on GFM functional specifications.
- Article
(1711 KB) - Full-text XML
- BibTeX
- EndNote
Ongoing rapid growth of inverter-based resources (IBRs) in modern power systems leads to a significant loss of system inertia and short-circuit power, which is followed by several challenges such as voltage, frequency, and synchronisation instability (Milano et al., 2018). The need for newer technologies, such as grid-forming (GFM) technology, has become inevitable to maintain and improving power system stability. The recent rapid growth in wind generation, including offshore wind power (IEA, 2023, 2022; Barthelmie and Pryor, 2021), also fosters the rise in large-scale offshore wind power plants (OF WPPs). As part of the major power source, GFM converter control technology must be integrated into the WPPs to enhance power system stability.
Existing OF WPPs (or IBRs in general) are dominated by grid-following (GFL) converter control technology, which synchronises itself to the grid via phase-locked loops (PLLs) and follows the frequency and voltage reference of the grid while injecting a constant power via a controlled current. Consequently, GFL converters have several issues related to their synchronisation stability, transient responses, weak grid operation, and grid support functionalities, to name a few (Aljarrah et al., 2024). On the other hand, GFM converters can define internal voltage and frequency and can behave as a voltage source, as opposed to the current source behaviour of the GFL converters (Matevosyan et al., 2019), and enhance the overall stability of an interconnected system (Pattabiraman et al., 2018) as well as of an islanded system (Verbe et al., 2021). In light of this, the grid-forming feature is considered an attractive solution for OF WPPs. However, there is a lack of clarity as to what functionalities and performance requirements are expected of a GFM implementation in OF WPPs.
Studies are necessary to further understand the GFM control method's applicability to power grids. The most reliable form of such studies is via field tests. To have ample field tests of GFM control, GFM converters must be integrated into the grid, which requires grid code requirements defined by transmission system operators (TSOs). TSOs prepare the grid codes based on their experience while considering suggestions from different stakeholders such as original equipment manufacturers (OEMs), equipment vendors, and power plant developers. To provide ample relevant recommendations to the TSOs to draft such grid codes, OEMs, vendors, and developers require further field tests, thus making this entire process a circular chicken-and-egg problem, as termed by an ESIG (Energy Systems Integration Group) task force (ESIG, 2022). Our approach can break the chicken-and-egg cycle by proposing performance specifications for GFM OF WPPs, which are based on the knowledge of the current state-of-the-art, mandatory performance for GFM behaviour. Further, we suggest a set of optional performance specifications, which existing GFL or GFM OF WPPs could provide. We also propose a set of advanced performance specifications for GFM OF WPPs, which require significant hardware changes, technological development, and experience in the field. In order to test the performance specifications, we also propose rudimentary testing guidelines and provide an overview of emerging test setups.
The paper layout is as follows: Sect. 2 summarises the functional specifications of GFM converters provided by various TSOs and research institutes. Section 3 points out the capabilities and limitations of OF WPPs and adapts and reclassifies the GFM performance specifications for OF WPP applications. Section 4.1 summarises the GFM performance specifications and recommends tests to assess them, and Sect. 4.2 provides an outlook of different next-generation test benches that could be utilised for GFM functionality testing.
The key contributions of this paper are summarised below:
-
A review of GFM functional specifications is provided by 13 different sources.
-
Adaptation of different GFM characteristics and functional specifications for weakly connected OF WPPs and their classification into mandatory, optional, and advanced requirements is provided.
-
Formulation of different test recommendations for individual mandatory, optional, and advanced GFM functional specifications is provided.
All electric power generators connected to the power grids must comply with a set of performance requirements known as grid codes and should exhibit specific performance for different testing requirements for various scenarios. For novel IBRs such as WPPs, battery energy storage systems (BESSs), and photovoltaic (PV) solar generation, specialised grid codes and performance requirements are needed, as general requirements are often not adequate or not applicable. Furthermore, different control methods could be applied for IBRs, such as GFL and GFM control methods, which exhibit different characteristics and dynamics during operation, thus reinforcing the need for specialised performance and testing requirements. This section reviews existing technical documents, white papers, and grid codes for GFM converters; discusses and critiques the provided specifications and requirements; and summarises them. For ease of readability, a summary of the proposed GFM requirements from these different technical documents, grid codes, and white papers is provided in Fig. 1.
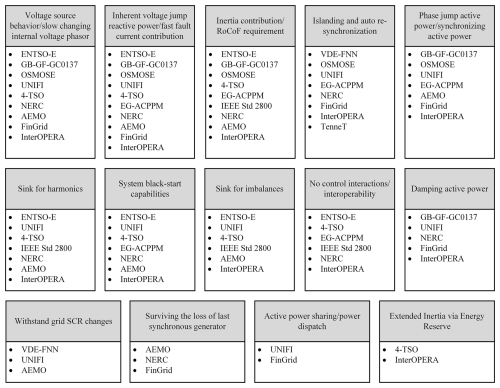
Figure 1Summary of GFM converter functional specifications as provided by different TSOs and research institutes.
Here, voltage source behaviour means that the voltage magnitude of the converter does not change significantly, and the converter provides a current response respective to the demand while maintaining the internal voltage phasor. Voltage jump reactive power/fast-fault current means that the converter inherently (not based on measurements) provides a reactive-power response to any grid voltage jump events or an inherent current response to faults based on the physics of the system. Inertia contribution/rate of change of frequency (RoCoF) relates to the case where any change in system frequency should be inherently reacted upon by the converter with an inertial response. Islanding and resynchronisation refers to the operation while generating enough power for the auxiliaries and maintaining an alternating current (AC) grid, and resynchronisation refers to re-establishing a stable connection to the grid after the islanding event clear-out. This differs from stand-alone operation or operation following the loss of the last synchronous generator. Phase jump/synchronising active power relates to the transient stability where the converter reacts to a grid phase jump with active power in an attempt to retain synchronism. Sink for harmonics implies that GFM converters should not inject any harmonics and should try to absorb grid harmonics whenever possible. Black starting relates to the ability to completely start the grid by energising the offshore cables, the transformers, and other passive-power-system components. Sink for imbalances indicates the requirement of withstanding or riding through an unbalanced fault or phase imbalance in large loads. Interoperability suggests that GFM converters should not have control interactions with other converters or active devices in the power system, irrespective of the vendor who supplies such converters or other devices. Damping active power refers to an active-power response to disturbances to avoid system oscillations. Withstanding grid short-circuit ratio (SCR) changes and surviving the loss of the last synchronous generator involve operating the GFM converter over a large range of grid conditions ranging from strong grid to weak grid to defining the grid, including the shifts between different grid strengths given by the grid SCR. Active-power sharing between GFM converters, although implicit, must be explicitly defined and classified as a requirement for converter-dominated power systems where power sharing and interoperability are crucial. Extended inertia refers to enhanced inertial characteristics that could be achieved in a GFM converter with the aid of an energy reserve device. These definitions are based on the definitions provided in the documents reviewed in this paper; however, a generalisation is made for the definitions. Thus, no specific document has been cited here. Various interpretations and an expansion of these definitions can be seen in the documents reviewed in this paper, and such distinctions are made in each of the following sub-sections if necessary.
2.1 ENTSO-E's technical report (ENTSO-E et al., 2017)
The European Network of Transmission System Operators for Electricity (ENTSO-E) published a technical report, High Penetration of Power Electronic Interfaced Power Sources and the Potential Contribution of Grid Forming Converters (HPoPEIPS), in 2017 (ENTSO-E et al., 2017). This technical report points out the stability issues/challenges for modern power systems such as reduced system inertia, short-circuit levels, and system split and other conventional issues such as rotor angle stability and voltage stability. Referring to GFM converters as a potential solution to tackle such problems, a set of performance specifications is proposed for GFM converters to enhance the system stability, which includes system voltage creation, fault current contribution, a sink for harmonics and imbalances, an inertia contribution, and prevention of control interactions. However, no constraints on the timescale have been placed on these performance specifications, which can result in ambiguity in the GFM implementation for WPPs. In addition, there is also a lack of clarity regarding the physical limitations that can impact the GFM nature of the WPPs. For example, according to the report since a GFM converter should, irrespective of its control technology, behave as a Thévenin-equivalent voltage source behind an impedance, GFMs need current limiting functionalities to protect the converter switches from over-current. This leads to crucial questions opening up an entire area of discussion and research: how should the current limiting be implemented without causing the converter voltage to change rapidly, and how should the current be prioritised during such events? Furthermore, a GFM converter is required to be a sink to harmonics for frequencies below 2 kHz. However, the technical report also identifies the underlying challenge behind this requirement: available headroom is required to inject harmonic current or provide harmonic damping during steady state.
2.2 NG-ESO's best-practice guide and GBGF specifications (NG-ESO, 2021, 2023)
The National Grid Electricity System Operator (NG-ESO) working group consultation specifies GFM requirements such as internal voltage source behaviour, phase jump active power, voltage jump reactive power/fast-fault current contribution, damping active power, inertial contribution, and a RoCoF requirement (NG-ESO, 2021). Black starting is not listed as a requirement for GFM converters; however, generation sources providing black-start services must have GFM functionality during operation. A supporting document – Great Britain Grid Forming (GBGF) (NG-ESO, 2023) – provides some updates on the operational design limits, such as phase jump limits, active-power transients, and minimum reaction time to phase jumps with no mandates for converter oversizing. Further, NG-ESO (2021) also prescribes similar tests, including RoCoF, phase jumps, fault ride through (FRT) and fast-fault current injection, three-phase faults followed by islanding, and converter power dynamics in response to grid frequency modulation based on network frequency perturbation (NFP) plots. Voltage source behaviour is considered a central element of GFM control, which is aided by the need for a physical reactor and no virtual impedance/admittance in GFM in the preceding document (NG-ESO, 2021). This requirement was later removed from the succeeding document (NG-ESO, 2023), thus facilitating the current correction/limiting via virtual admittance in the internal voltage loop of the GFM converter.
With some consideration of the initial operating conditions, energy availability, and mechanical design, these GFM performance specifications could be imposed on OF GFM WPPs.
2.3 VDE-FNN GFM guidelines (VDE-FNN, 2020)
The German technical regulator VDE-FNN (Verband der Elektrotechnik, Elektronik und Informationstechnik, Forum Netztechnik/Netzbetrieb) prepared guidelines that provide general requirements for stable system operation of a power system; however, it does not specify the technical requirements (VDE FNN, 2020). The document defines a list of testing scenarios to validate the GFM performance specifications. For example, tests on grid voltage phase and magnitude step, grid RoCoF response, harmonics and sub-harmonics, the negative-sequence current in the grid and its effect, islanding, and the grid short-circuit ratio (SCR) change, to name a few. These test cases are similar to the requirements proposed by the previous two papers, ENTSO-E et al. (2017) and NG-ESO (2021). The International Council on Large Electric Systems (CIGRE) also proposes test frameworks for high-voltage direct current (HVDC) and flexible AC transmission system (FACTS)-based GFMs, which includes a collaborative approach between TSOs and OEMs to GFM testing. The general recommendations of GFM requirements, test scenarios, and test frameworks are for HVDC systems and DC-connected power park modules (PPMs), which can also be adapted for OF WPPs with AC network connections. Further details on the testing specifications provided by VDE-FNN are presented in Sect. 4.
2.4 OSMOSE BESS converter requirements (OSMOSE, 2021)
Optimal System-Mix Of flexibility Solutions for European electricity (OSMOSE) – a European project led by the French TSO RTE and joined by 6 European TSOs and 33 partners – presents different GFM requirements for power converters used in BESSs (OSMOSE, 2021). These requirements comprise voltage source behaviour with a slow-changing internal voltage phasor, power-based synchronisation, the ability to withstand RoCoF, under-voltage ride through (UVRT), fast-current injection, and islanding capability, while stressing that energy headroom availability is crucial for GFM operation. Thus, OSMOSE requirements in general align with the National Grid ESO (NG-ESO, 2021, 2023) requirements, with a primary focus on applications to BESSs.
OSMOSE defines four types of GFM units with different capabilities apart from the core capabilities discussed earlier. The enlisted GFM unit types indicate that converter performance governs the GFM behaviour, not its control design.
- a.
Type I GFM unit. Capable of standalone operation, it provides system strength(V–Q support) and fault current (). Here, In is the nominal current capability respective to the rated power of the GFM inverter-based-resource (IBR) unit, and Ifault is the fault current capability of the GFM IBR unit.
- b.
Type II GFM unit. This is a type I GFM unit with synchronising power.
- c.
Type III GFM unit. This is a type II GFM unit with inertial response
- d.
Type IV GFM unit. This is a type III GFM unit with fault current (Ifault>2In).
2.5 UNIFI consortium GFM IBR specifications (Kroposki et al., 2022)
The universal interoperability for grid-forming inverters (UNIFI) consortium provides GFM requirements for IBRs inclusive of various generation sources, namely, solar PVs, BESSs, WPPs, static synchronous compensators (STATCOMs), and fuel cells, to name a few (UNIFI Consortium, 2022). The specifications are classified as follows:
- a.
Universal.
- i.
Normal operation. Autonomous grid support based on local measurement, the ability to dispatch, positive damping to voltage and frequency oscillations, power sharing among generators, weak-grid operation and stability enhancement, and maintaining voltage balance must be present during normal operation.
- ii.
Abnormal operation. FRT, voltage source behaviour during asymmetrical faults, frequency response, inherent power responses to voltage magnitude and phase shifts, and islanding and resynchronisation must be present during abnormal operation.
- i.
- b.
Additional. Black-start capability, reduction in voltage harmonics, cyber-secure communication, and secondary control of voltage and frequency are additional features.
Here, it is unclear if the inclusion of cyber-secure communication in the additional requirement implies the need for communication for grid-connected operation, synchronisation, and power sharing. It is a general understanding in the technical field that GFM converters need to be able to operate, fulfilling the grid code requirements without the need to communicate with each other. However, secure communication between the power plant controller (PPC) and the wind turbine generators (WTGs) is necessary, irrespective of the control method.
2.6 Requirements by 50 Hertz, Ampiron, TenneT, and Transnet-BW (4-TSOs) (50Hertz et al., 2022)
Four German TSOs, namely 50 Hertz, Ampiron, TenneT, and Transnet-BW, collaborated to summarise mandatory, additional, and optional features of GFM converters (50Hertz et al., 2022). Highly influenced by the HPoPEIPS report (ENTSO-E et al., 2017), this report classifies GFM capabilities as the following:
- a.
Mandatory. Voltage source behaviour, fast-fault contribution, inertia contribution, control interaction prevention, and converter stability are mandatory.
- b.
Additional. Sinks for harmonics and for phase imbalance and additional inertia by an extended energy reserve are additional.
- c.
Optional. Black-start capability is optional.
The collaboration between four different European TSOs in this paper sends a strong message that GFM IBRs are necessary for system stability. It also shows that TSOs are prepared to collaborate, contribute, and aid in the technological and policy-level development of GFM IBRs.
2.7 Expert group on AC PPMs (EG-ACPPM, 2023)
In a report presented to the Grid Connection European Stakeholder Committee (GC ESC), the expert group on AC PPMs (EG-ACPPM) defined basic characteristics for GFM PPMs, which include the creation of system voltage and the contribution to fault level, inertial contribution, and control robustness and stability (EG-ACPPM, 2023). The expert group report notes that the PPMs should be able to operate according to different network requirements such as active and reactive power provision, withstanding a blackout of 24 h, black-start capabilities (optional, but black-start-capable PPMs must be grid forming, which is adapted from NG-ESO, 2021, 2023), islanding capabilities, and resynchronisation, to name a few. However, the report adds that the impact on PPMs in terms of technological and hardware changes to provide the GFM services must be evaluated. The EG-ACPPM also notes that the ability to withstand UVRT and RoCoF is essential for GFM PPMs, and studies on over-voltage ride through (OVRT), phase jump active power, and voltage jump power are also necessary. In addition to the GFM requirements, the EG-ACPPM summarises generation-source-specific capabilities and limitations to provide GFM response for PPMs and also includes a list of needed measures to utilise the capabilities. The capabilities and limitations presented in the report are reviewed in Sect. 3.1 for OF WPP applications.
2.8 ESIG task force (ESIG, 2022)
The ESIG task force (ESIG, 2022) defines the GFM operational requirement and the lack of field knowledge and technological know-how as a chicken-and-egg problem, as one requires the other. The task force proposes an iterative process to break the cycle by defining a target system to perform tests to determine system needs and functionalities and to implement them after rigorous field testing, quality checks, and monitoring. Feedback from these steps updates the definition of the target system. ESIG defines the system needs as synchronisation, voltage and frequency regulation, damping, protection, restoration, capacity, and energy availability. It further proposes the operational requirements of GFM converters based on these system needs. A summary of tests applicable to both GFM and GFL converters is also presented in the document, which can help define different testing requirements for such converters for OF WPP applications. Although this report does not specifically mention or summarise GFM performance specifications itself, it provides a practical roadmap that could be followed to define such specifications.
2.9 IEEE standard 2800–2022 (IEEE 2800, 2022)
Institute of Electrical and Electronics Engineers (IEEE) standard 2800-2022 (IEEE 2800, 2022) defines requirements for inverter-based generation in general without specifying the converter control class, namely GFL or GFM. Some of these requirements, such as reactive power and voltage responses, active power and frequency responses, power quality, and several test requirements, could be adopted readily for GFMs used in OF WPPs. The standard highlights an essential point about the interoperability of different power system components, which will be crucial for the operation of the IBR-dominant power grids. The standard further points out that a crucial objective for GFM IBR should be to utilise the full capabilities of IBRs in the face of evolving power systems rather than to attempt to merely reproduce the behaviours of synchronous machines.
Although the standard generally discusses IBRs, the insights into the interoperability of IBRs and the IBR testing requirements it provides are crucial for adaptation to GFM OF WPPs. As is the case for the other documents reviewed – where GFM specifications are defined as either agnostic of the generation sources or for specific generation sources that are not OF WPPs – we have reviewed and adapted the specifications and testing suggestions for GFM OF WPP uses. Similarly, we adapt some generic IBR requirements and testing suggestions for GFM OF WPPs in this paper.
2.10 NERC GFM operational requirements (NERC, 2023, 2021)
The white paper by the North American Electric Reliability Corporation (NERC) provides several operation requirements, including a constant or nearly constant internal voltage phasor during the transient and sub-transient time frame, operation in low system strength, grid frequency and voltage stabilisation, resynchronisation, FRT, fault current contribution, and optional black-start capabilities (NERC, 2023). However, since the US grids have a dominant solar PV generation (18 % of total utility-scale renewable energy generation in 2023 was from solar PV plants; US-EIA, 2023), NERC's GFM converter requirements significantly reflect the capabilities of the DC buffer/storage devices seen in solar PV plants. A previously published document from NERC (NERC, 2021) also summarises the GFM capabilities for general operation. These include the operation of GFM in low system strength, frequency and voltage stabilisation, small signal stability and power system oscillation damping, resynchronisation (following unintentional islanding), FRT and fault current contribution within the hardware limits, and black-start capabilities for system restoration. This document addresses the numerous challenges facing GFM converters, namely the technological and resource capabilities, grid resilience, available energy headroom, and the possibility of encountering newer stability challenges following the massive integration of GFM converters in the future. The report also provides an overview of different GFM converter control methods and recommends that GFM control performance assessment be based on their performance and not on the control strategy, which was also pointed out by the Electric Power Research Institute (EPRI; EPRI, 2023).
2.11 Voluntary GFM specifications by AEMO (AEMO, 2023, 2024)
The Australian Energy Market Operator (AEMO) developed voluntary specifications for GFM converters and classified them into core and additional capabilities as follows (AEMO, 2023):
- a.
Core capabilities. These are voltage source behaviour, the response to voltage magnitude and phase changes, improved frequency-domain dynamics with low impedance around system fundamental frequency, inertial response (with a suitable energy buffer via storage and power headroom via plant oversizing), and surviving the loss of last synchronous generator.
- b.
Additional capabilities. These are over-current capability, black starting, and power quality improvement.
The voltage source behaviour of GFM converters, as defined by AEMO, requires GFM to have a constant internal voltage phasor that is constant in a short time frame; i.e. during the sub-transient phases following a grid event, the internal voltage phasor of the GFM converter should change slowly or stay constant. The initial response of the GFM converters following a disturbance should start within a few milliseconds. This requirement is also consistent with the recommendations of GBGF (NG-ESO, 2021) and FinGrid (FinGrid, 2023); the former was discussed earlier in this paper, and the latter is discussed in the following section.
Further, a subsequent document by AEMO on the core requirement test framework was released in 2024 (AEMO, 2024), where a set of simulation-based test methods are provided to assess the GFM capability of inverters as defined in its predecessor document (AEMO, 2023).
2.12 Grid forming for BESSs by FinGrid (FinGrid, 2019/2020, 2023)
The TSO of Finland, FinGrid, developed grid codes for energy storage systems, typically BESSs (FinGrid, 2019/2020). Following the grid code specifications, specific study requirements were formulated for storage systems larger than 30 MW connected at 110 kV or higher (classified as a type D BESS) (FinGrid, 2023). FinGrid provides functional requirements, active-power-control and frequency control requirements, voltage and reactive-power-control requirements, modelling requirements, and test requirements, a classification distinct from the other grid codes and performance specifications reviewed in this paper. The GFM converters are not allowed to limit the current below its capacity artificially, and they must provide GFM responses up to their rated capacity with no obligation to do so above or beyond their physical capabilities and available energy. GFM capabilities are required of the type-D BESSs within the entire range of its state of charge (SoC), and grid support must be maintained even during current-limited operation. Further, type D GFM BESSs are not allowed to operate in GFL mode when connected to the network, should resist fast changes in the internal voltage phasor in response to phase jumps, and provide near-instantaneous active and reactive power responses for frequency and voltage support in a sub-transient time frame, with an initial response within a few milliseconds and a full response within 10 ms, suggesting the need for significant damping. Other GFM requirements involve a seamless transition between islanded and grid-connected modes, operation in constant active-power mode and frequency-power droop mode with GFM control, and operation in constant-reactive-power mode and voltage-reactive power droop mode with GFM control. Various simulation tests that FinGrid defines for GFM converters will be discussed in Sect. 4.
2.13 InterOPERA
A European Union-funded project on interoperability of multi-terminal multi-vendor HVDC systems was initiated in 2023, which developed functional specifications for grid forming in HVDC converter stations and DC-connected PPMs in 2024 (InterOPERA, 2024). This functional requirement document mentions core/mandatory and optional features/functions of OF WPP-based GFM converters integrated into HVDC terminals. This document prepares a roadmap for a “demonstrator”, which studies the interoperability of multi-vendor, multi-terminal offshore HVDC interconnection systems, with PPMs consisting of GFM or GFL converter control, and explores vast areas such as interoperability, DC-FRT, and different use cases. This document considers the inherent reactive power capability and fast-fault current capability of GFM converters to be the same requirement/capability. Further, the document highlights that only capabilities unique to grid forming need to be classified as core capabilities. Thus, three categories of GFM performance are defined as follows:
- a.
Mandatory requirements. These constitute the core requirements that could be fulfilled with a GFM converter only and include self-synchronisation, phase jump active power, inertial active power, inherent reactive power, and positive-damping power.
- b.
Optional requirements. These constitute advanced GFM capabilities, e.g. black starting, and those capabilities achievable by GFL controls, e.g. a sink for voltage unbalance and a sink for harmonics.
- c.
Ability to withstand. These constitute the converters' abilities to withstand a large SCR change, a phase jump, RoCoF, and temporary islanding.
The GFM functional requirements reviewed in the previous section present multiple perspectives of the TSOs, expert groups, task forces, research institutes, and standards committees. Such requirements and specifications were defined for either generation-source-agnostic GFM converters or GFM converters used in resources such as BESSs. An important consideration necessary to adapt such functional specifications for GFM OF WPP applications (or any other generation source) is to understand the physical capabilities and limitations of the generation source (EG-ACPPM, 2023). As the physical capabilities and limitations of OF WPPs impact the GFM performance of their converters, this section explores such capabilities and limitations and adapts the aforementioned GFM specifications for OF WPP applications. The reclassification of the GFM functional specifications into mandatory, optional, and additional categories is also based on the same.
3.1 Physical capabilities and limitations of GFM OF WPPs
Significant distinctions between an onshore wind power plant and an offshore wind power plant are in the grid-connection method and power evacuation (National Grid, 2023). The physical limits of the power evacuation system, as mathematically shown by the SCR, limit the GFM performance of the OF WPP, along with its short-circuit performance and overloaded power evacuation. Further, for advanced GFM capabilities such as black starting, the vast evacuation network and, in some cases, the necessity of including active devices such as STATCOM and synchronous condensers for stability and flexibility pose a significant challenge.
OF WPPs with type IV WTGs are connected to the grid via long AC transmission lines, which have large impedances and low SCRs, or via HVDC cables (Johansen, 2020). The generation source itself is operated at its maximum power capacity or close to the maximum power capacity for the available resource at any instant, via maximum power point tracking (MPPT) algorithms. Thus, OF WPPs have limited headroom in their generation, restricting the GFM operations such as phase jump active power response and inertial response. The maximum overload capacity of the power-electronic switches of the converter is significantly low compared to synchronous machines, for example, around 1.11 pu of the current injection (Gomes Guerreiro et al., 2023). Even when wind is available and within the mechanical capacity of the WTGs, any overloading beyond this can significantly stress and damage the power-electronic components of the WTG over its lifetime (Erlich et al., 2009). Further, DC link energy availability (Erlich et al., 2009) also imposes a significant limitation on power transfer during transients and thus also imposes limitations on the GFM operation.
However, suppose that the WTGs are utilised to curtail the available power for any given wind conditions. In that case, this provides some headroom for the GFM converter to react to any system events and provides the required GFM response, such as an inertial response, phase jump active power response, or a fault current contribution without overloading the power electronic switches (Lyu and Groß, 2024). This solution requires no hardware changes and thus can be achieved with simple software changes; however, it still imposes significant financial constraints on the generators, as the curtailed power will not be transferred to the grid. Furthermore, this also implies that the GFM behaviour of OF WPPs depends highly on the initial operating point and energy availability.
EG-ACPPM suggests using additional storage capacity at the DC link, fundamentally re-engineering the converter control and using additional chopper capacity, over-dimensioning, and enhanced power headroom to enhance the GFM performance of the type IV WTGs (EG-ACPPM, 2023). Although these solutions improve the technical capabilities in terms of the GFM requirements of OF WPPs, it must be noted that this implies significant hardware and control changes, thus putting significant financial constraints on the manufacturers and developers, which can lead to a hike in the overall cost of the technology. Considering this, several TSOs (as discussed in the previous section) do not impose any hardware changes/additions to enhance GFM performance, which helps manufacturers investigate low-cost solutions to the GFM requirements. Therefore, any suggestions/conclusions regarding the need for significant hardware modifications/additions presented in this paper should be considered a potential exploratory path for further research and not strict recommendations to implement them.
3.2 Classification of GFM performance specifications
Giving the GFM requirements in Fig. 1 due consideration and also taking into account the physical limitations of the WTGs and their associated mechanical and electrical components, a reclassification of the GFM performance specifications is performed in this section. An overview of the reclassification definition of mandatory, optional, and advanced GFM requirements is presented in Fig. 2.
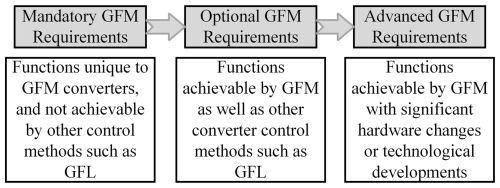
Figure 2Reclassification of GFM functionalities: the definitions of the requirement/specification categories.
3.2.1 Mandatory performance specifications
The GFM performance requirements, which are unique to GFM converters and can only be achieved by GFM converters, are included in this category. The performance specifications such as voltage source behaviour, synchronising active power, damping active power, inertial contribution, voltage support, FRT capabilities, control robustness, and withstanding grid SCR changes and RoCoF are part of a majority of the technical documents reviewed in Sect. 2. These requirements are the core capabilities expected of a GFM converter for any source, whether it be a BESS, HVDC, or WPP. OF WPPs especially have some restrictions in and limitations on their inertial properties and the overload capacity needed for requirements such as FRT capabilities, withstanding RoCoF, and damping power. However, with certain power boost features of the new OF WPPs, it is possible to explore these capabilities to some degree. Further, these requirements define the grid-forming behaviour at the most basic level and provide some necessary variations from and updates to the grid-following behaviour. They also provide some similarities to some of the properties of the synchronous machines primarily sought by IBRs. Thus, they are classified as mandatory requirements of GFM OF WPPs. Further, some requirements are classified as mandatory due to the nature of the GFM converters' responses to them and the use of the same/similar tests to assess them, namely, inertial response, RoCoF, and phase-jump/damping/synchronising active power.
3.2.2 Optional performance specifications
The optional performance specification category involves performance requirements that could be an additional service to be provided by GFM converters, which other converter control methods such as V/F control or GFL control could provide. Typically, harmonics can be avoided by properly tuning filters or by advanced techniques such as active filtering (Kocewiak et al., 2023). GFL converters can also cancel them and thus are usually classified as optional GFM requirements in the literature (InterOPERA, 2024). Although it is necessary for GFM converters to not only avoid injecting unnecessary harmonics but also offer a sink for the harmonics arising from the grid, this requirement does not affect the core grid-forming behaviour and could be achieved with GFL converters; thus it is placed in the optional category of GFM requirements.
Furthermore, the requirement concerning the ability to withstand imbalances and to provide a sink to grid imbalances by riding through unbalanced faults requires dedicated positive- and negative-sequence controls. These control realisations are not presented in this paper as they are not within the scope of the study, namely, the study of the dynamic behaviour of various OF WPP-based GFM converters versus the performance specifications. This requirement is also not a core capability of GFM and can be realised in other primitive control methods such as GFL. Hence, it is classified as an optional requirement.
3.2.3 Advanced performance specifications
To provide extended inertia services, GFM converters need to be equipped with a reinforced DC bus, namely an energy buffer in the form of a flywheel energy storage system, a supercapacitor, or a battery (Rokrok et al., 2022). This technological extension increases the financial burden on OEMs, the inherent risks, and the operation and maintenance (O&M) requirements. Taking the consequences into consideration and acknowledging the need for extended inertia, this requirement is placed in the optional requirement category.
Islanding and auto-resynchronisation of OF GFM WPPs also present significant challenges, especially in the absence of a local load. The available energy following the islanding must be dissipated to the auxiliaries, to the local loads, or via the DC chopper. In reality, the DC chopper has a limited capacity (Xu et al., 2021), the auxiliary consumption is typically low, and the local loads are absent. Thus, maintaining steady open-circuit-rated voltage during the islanding is challenging. Further, the auto-resynchronisation following the islanding can lead to a significant power surge as the phase angle between the point of common coupling (PCC) and the converter could differ. Achieving momentary islanded operation and auto-resynchronisation following the islanding potentially needs further studies on control strategies and hardware changes. Thus, this capability of GFM converters is categorised as an advanced requirement.
Similarly, following the loss of the last synchronous generator in the grid, the GFM converter must autonomously run the entire grid by providing voltage and frequency set points. This situation arises in a modern power system when most conventional synchronous generators are replaced by inverter-interfaced generation, and GFM IBRs run the power system. The research field has some experience in running micro-grids with GFM converters (Musca et al., 2022); however, the existing knowledge and experience are not adequate for a large power system, as new challenges might arise with a high share of GFM IBRs in the system. As more studies, knowledge, and experience are required, assessing the fulfilment of this requirement is challenging. Considering the underlying challenges and lack of available studies on the subject matter, this requirement is categorised as an advanced requirement.
Black starting a grid involves the gradual energisation of various power system components, such as the transformer, transmission lines, and substations, thus building up the system voltage and a steady, normal power flow. This area needs further research and field tests for OF WPPs as well as for other IBRs. Thus, achieving a black start with an OF WPP with GFM control is classified as advanced performance.
The assessment of GFM capabilities of OF WPPs requires appropriate tests. The tests range from simulation-based tests for preliminary studies; hardware-in-the-loop (HiL) and software-in-the-loop (SiL) tests for more advanced studies involving equipment prototyping, verification, and use of real control code for the same; to novel test benches for vendor and manufacturer-specific tests, developments, and studies. Overall, the starting point for these tests is simulation-based tests, which are scalable and flexible for multiple operating points and, considering accurate model development and parameterisation, are reliable for a general idea of the operation of the device under scrutiny. This section suggests simulation-based tests for the GFM specifications defined and classified in the paper. Further, an insight into emerging test benches such as HiL, SiL, and vendor/manufacturer-specific test benches is provided.
4.1 Simulation-based tests of GFM specifications
The test framework of the GFM performance is a challenging topic to undertake as it requires a deep understanding of the GFM converters' performance requirements and capabilities and significant practical experience with specific generation sources. Most existing testing recommendations are for generators as a whole or for a specific generation type with no specific control method (GFL or GFM). Only a few TSOs have defined the testing requirements for GFM converters, which are also brief. Merely following the existing testing requirements to build a test framework for OF GFM WPPs might not be relevant. Thus, this paper attempts to review the different test requirements provided by different grid codes and prepares relevant simulation-based test requirements for OF GFM WPPs. The goal of this section is not to numerically specify the test conditions but to provide a practical perspective on the test severity that could be imposed on an OF GFM WPP simulation model.
Voltage source behaviour or a slow-moving internal voltage phasor is the core of GFM control and is required by many GFM performance specifications. The voltage source behaviour could be assessed by passive-impedance behaviour in the 5 Hz–1 kHz range (NG-ESO, 2019). Further, constant or nearly constant internal voltage phasor in the sub-transient time frame could be attributed to the good voltage source behaviour of the GFM converter. Thus, the terminal voltage of GFM converters should be constant or nearly constant in the transient and sub-transient time frame (<5–10 ms following the disturbance). The disturbance could be a grid phase jump or a three-phase fault.
Energinet – the Danish TSO – has defined technical regulations for WPPs above 11 kW, which are devoted to neither specific converter control methods of the WPPs nor specific onshore or OF WPPs (Energinet, 2016). Nevertheless, the operational requirements and tolerances for WPPs during different grid events at PCC are defined, and these could be adapted for simulation-based tests on GFM converters. In contrast to the NG-ESO requirements of 30° phase jump tests and ±5 % grid voltage jump tests for electrical metallic tubing (EMT) simulation models (NG-ESO, 2024), Energinet specifies tolerance to a 20° phase jump for 80–100 ms and ±20 % grid voltage jump for 0.5 s for WPPs with capacity higher than 25 MW. It must be noted that tolerance of smaller phase jumps could be achieved by a GFM converter within its physical operation limits. However, for larger phase jumps, especially when the GFM converter is operating near the maximum power, the phase jump can lead to instability, as shown in Fig. 3. Thus, while defining phase jump test requirements for GFM converters, the operating points, the available energy, and the energy headroom must be considered.
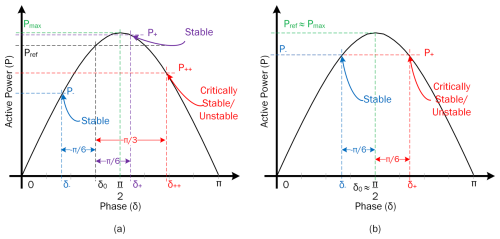
Figure 3P–δ curves (illustration only) for large phase shifts: (a) for operating points below the rated power (Pref<Pmax) and (b) for operating points close to the rated power (Pref≈Pmax).
Energinet requirements also require WPPs to ride through 150 ms phase–ground, phase–phase–ground, and three-phase bolted faults for the WPPs (Energinet, 2016), which could be adapted for an OF GFM WPP; however, initial studies on the FRT capabilities for GFM must be exploratory. However, a more crucial point to address for GFMs regarding their fault response is the inherent current capability they are required to possess. In response to any fault, the GFM should inherently start injecting a fault current within its operational and hardware limits, without relying on external measurements and control. The response start time needs to be within the sub-transient time frame and neither related to nor dependent on the measurement and control devices and their delays.
OF WPPs are characterised by weak grid connections with the SCR at PCC, typically below 2, which change dynamically during operation. Thus, a GFM OF WPP should be able to operate in a wide range of grid SCRs and withstand changes in these SCR values. Quantification of the specific values of SCR levels within which the OF GFM WPP is required to stay stable is not defined in this paper; however, it is recommended that an operating point sweep and stability analysis for a range of SCR values between its maximum and minimum be performed to ensure that the GFM converter can provide stable operation within that range.
In the grid code documents reviewed here, the requirements for islanding and resynchronisation for GFM converters are not clearly stated, as is the case for other operational requirements. Topics such as islanding, resynchronisation and black starting and grid events such as the loss of the last synchronous generator in the grid are challenging to study and assess in simulation-based tests, as they require extensive prototype and field testing. TenneT Annex C2.300 (TenneT, 2023) provides an islanding requirement of 150 ms for DC-connected PPMs, and InterOPERA plans to adapt this requirement and further explore the possibility of 300 ms islanding without enforcing any significant hardware changes on the PPMs (InterOPERA, 2024). However, both these requirements/proposals are for HVDC-connected PPMs following a DC fault and temporary blocking of the HVDC converters. They may not apply directly to AC-connected OF WPPs under their existing definition. Although OF GFM WPPs could withstand shorter islanding situations on the order of a few tens of milliseconds, resynchronisation strategies might be required for longer islanding situations. Following a longer islanding situation, the converter phase and the grid phase can vary significantly. Without a proper resynchronisation strategy, the resynchronisation attempt can resemble a large grid phase shift, leading to instability. This is illustrated in Fig. 4. In some cases due to the dynamic nature of the phase angles, the phase difference between the converter and the grid at the time of resynchronisation could also be aligned. However, this can still lead to issues such as over-burning of the DC chopper and large mechanical stress on the mechanical structure of the wind turbines (Xu et al., 2021).
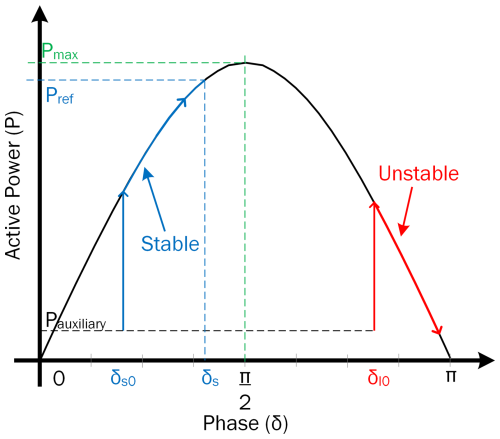
Figure 4P–δ curves (illustration only) for short islanding (blue) and long islanding (red) cases followed by an auto-resynchronisation attempt. More details on islanding of GFM WTGs is provided in Ghimire et al. (2024).
A summary is prepared for the proposed mandatory and optional GFM requirements for OF WPPs, alongside the simulation-based tests to evaluate such requirements. It is presented in Table 1.
4.2 Emerging test benches for GFM tests
Traditionally, for WTGs and other equipment, assessment of grid compliance capabilities at the equipment level is performed using a full-scale prototype connected directly to the grid. A number of tests can be performed at this level, ranging from active and reactive power (PQ) capability curves, harmonics, and flickering to under-voltage ride-through (UVRT) and over-voltage ride-through (OVRT) using a container test setup. Nonetheless, this testing method can also offer many challenges in terms of testing limitations, safety, and limited freedom to change grid conditions (e.g. frequency, SCR, and voltage).
Certain GFM requirements cannot be easily tested via traditional methodologies, such as equipment prototype turbines connected directly to the system or connected at the plant level (Gomes Guerreiro et al., 2024). Capabilities such as black starting, island operation, inertia response, withstanding RoCoF, etc., can be challenging to test for the first time on equipment/plants connected directly to the power system. Additionally, it is important to validate models for these additional capabilities. Thus, advanced testing methods in a controllable environment, such as power hardware in the loop (PHiL) with grid emulators connected to either subsystems or the prototype equipment, are emerging as important tools to assess GFM performance. Standardisation of such test benches is also ongoing in technical fora, such as the International Electrotechnical Commission (IEC) 61400-21-4 standard (IEC, 2023).
Various next-generation PHiL test benches have been proposed in Curran et al. (2022), Neshati et al. (2023), Li et al. (2023), and Gevorgian et al. (2023). These test benches use only a few selected components; the rest of the equipment is represented by employing novel modelling and emulation techniques for the missing hardware parts. These test benches consist primarily of the converter hardware and control system, which are connected to separate inverter systems capable of emulating either the generator and grid side or the grid side only. For example, Neshati et al. (2023) present a novel test rig with generator and grid-side emulators that can realistically emulate GFM capabilities. The generator-side emulator communicates with a real-time simulation of high-fidelity models of the WTG and aerodynamic components, which include wind field, turbulence, and so on. The grid simulator uses an inverter-based control of the grid voltage to allow the simulation of basic features such as grid FRT and of advanced features such as dynamic impedance emulation, phase jumps, voltage steps, RoCoF, and harmonic control.
On the other hand, the use of grid emulators (Li et al., 2023; Hans et al., 2022, 2023) on tests performed at the equipment level is also rising, where instead of connecting the equipment directly to the system, grid emulators are connected to the equipment. This allows for a larger variety of tests and more controllability of testing conditions, as the grid emulator can respond to any desired set points and operating conditions.
This paper reviews and summarises different grid codes and white papers and technical documents on GFM functional specifications, operational requirements, and testing requirements. It also adapts the available functional specifications and operational requirements for OF GFM WPPs. It reclassifies them as GFM functionalities unique to the GFM (mandatory requirements), GFM functionalities achievable by other converter control methods (optional requirements), and advanced GFM functionalities that require hardware modification or significant technological advancements (advanced requirements). This paper also reviews various testing requirements for various generation sources and adapts them for OF GFM WPPs.
This paper also presents an overview of various other applications of GFM converters and recommendations for adapting the control requirements and functional specifications for these applications. Furthermore, a short review of the existing next-generation test benches is provided, with an outlook to their potential application for testing the capabilities of GFM converters for various generation sources.
It was observed in the rigorous literature review that the initial operating point for the GFM functional specifications is not defined. The behaviour of GFM OF WPPs depends on their initial operating point and curtailment status; thus, the GFM standards need to specify these specifications for various initial operating points. Further, the standards need to consider the limitations of OF WPPs and the limitations of the switching devices. Finally, GFM specifications and requirements in grid codes must be technology agnostic. Amidst the lack of literature, namely technical reports and performance specification recommendations of GFM converters for OF WPP application, this paper thus provides a framework to define and reclassify GFM requirements, recommend tests for evaluating such requirements, and also provide an overview of advanced test setups and how they could be utilised for the assessment of GFM behaviour.
No data sets were used in this article.
SG wrote the original draft. GMGG wrote Sect. 4.2 and provided feedback on the rest of the sections. KVK and KHJ provided suggestions for recommended tests in Table 1 and also supported the revision of the paper. KHJ and EDG provided guidelines, prepared the plans for the research as part of the funding application, and helped formulate the paper contents and goal. GY and XW contributed to the guidance and revisions.
The contact author has declared that none of the authors has any competing interests.
Figures and values presented in this paper should not be used to judge the performance of Siemens Gamesa (SGRE) technology, as they are solely presented for demonstration purposes. Any opinions or analyses contained in this paper are the opinions of the authors and are not necessarily the same as those of SGRE.
Publisher's note: Copernicus Publications remains neutral with regard to jurisdictional claims made in the text, published maps, institutional affiliations, or any other geographical representation in this paper. While Copernicus Publications makes every effort to include appropriate place names, the final responsibility lies with the authors.
This article is part of the special issue “Electro-mechanical interactions in wind turbines”. It is not associated with a conference.
This research has been supported by the Innovationsfonden (grant no. 0153-00256B).
This paper was edited by Amir R. Nejad and reviewed by four anonymous referees.
AEMO: Voluntary Specification for Grid-forming Inverters, Australian Energy Market Operator, https://aemo.com.au/-/ media/files/initiatives/primary-frequency-response/2023/ gfm-voluntary-spec.pdf?la=en&hash= F8D999025BBC565E86F3B0E19E40A08E (last access: May 2024), 2023. a, b
AEMO: Voluntary Specification for Grid-forming Inverters: Core Requirements Test Framework, https://aemo.com.au/-/media/files/initiatives/engineering-framework/2023/grid-forming-inverters-jan-2024.pdf?la=en (last access: October 2024), 2024. a
Aljarrah, R., Fawaz, B. B., Salem, Q., Karimi, M., Marzooghi, H., and Azizipanah-Abarghooee, R.: Issues and Challenges of Grid-Following Converters Interfacing Renewable Energy Sources in Low Inertia Systems: A Review, IEEE Access, 12, 5534–5561, https://doi.org/10.1109/ACCESS.2024.3349630, 2024. a
Barthelmie, R. J. and Pryor, S. C.: Climate Change Mitigation Potential of Wind Energy, Climate, 9, 136, https://doi.org/10.3390/cli9090136, 2021. a
Curran, O., Guerreiro, G. M. G., Azarian, S., Martin, F., and Dreyer, T.: WTG Manufacturer's Experience with Subsystem and Component Validation for Wind Turbines, 21st Wind & Solar Integration Conference, October 2022, The Hague, the Netherlands – IET Conference Proceedings, 458–465, https://digital-library.theiet.org/content/conferences/10.1049/icp.2022.2812 (last access: May 2024), 2022. a
EG-ACPPM: Expert Group: Advanced Capabilities for Grids with a High Share of Power Park Modules, European Union Agency for the Cooperation of Energy Regulators, https://eepublicdownloads.blob.core.windows.net/public-cdn-container/clean-documents/Network%20codes%20documents/GC%20ESC/ACPPM/TOP.2.b._GC-ESC_EG_ACPPM_Report_version_1.00.pdf (last access: May 2024), 2023. a, b, c
Energinet: Classification: Public Technical regulation 3.2.5 for wind power plants above 11 kW, https://en.energinet.dk/media/5pbflmly/technical-regulation-325-for-wind-power-plants-above-11-kw (last access: May 2024), 2016. a, b
ENTSO-E, Wind Europe, Solar Power Europe, and T&D Europe: High Penetration of Power Electronic Interfaced Power Sources (HPoPEIPS), https://api.semanticscholar.org/CorpusID:139087166 (last access: May 2024), 2017. a, b, c
EPRI: Grid Forming Inverters: EPRI Tutorial, Electric Power Research Institute, Palo Alto, CA, https://www.epri.com/research/products/000000003002028090 (last access: May 2024) 2023. a
Erlich, I., Shewarega, F., Engelhardt, S., Kretschmann, J., Fortmann, J., and Koch, F.: Effect of wind turbine output current during faults on grid voltage and the transient stability of wind parks, in: 2009 IEEE Power & Energy Society General Meeting, July 2009, Calgary, Alberta, Canada, 1–8, https://doi.org/10.1109/PES.2009.5275626, 2009. a, b
ESIG: Grid-Forming Technology in Energy Systems Integration, High Share of Inverter-Based Generation Task Force, Energy Systems Integration Group, Reston, VA, https://www.esig.energy/reports-briefs (last access: May 2024), 2022. a, b
50Hertz, Ampiron, TenneT, and TransnetBW: 4-TSO Paper on Requirements for Grid-Forming Converters, https://www. netztransparenz.de/xspproxy/api/staticfiles/ntp-relaunch/ dokumente/zuordnung_unklar/grundlegende-anforderungen-an-netzbildende-umrichter/220504_-_4-tso_paper_on_requirements_for_grid-forming_converters.pdf (last access: May 2024), 2022. a
FinGrid: Grid Code Specifications for Grid Energy Storage Systems SJV2019, https://www.fingrid.fi/en/grid/grid-connection-agreement-phases/grid-code-specifications/grid-energy-storage-systems/ (last access: May 2024), 2019/2020. a
FinGrid: Specific Study Requirements for Grid Energy Storage Systems, https://www.fingrid.fi/globalassets/dokumentit/fi/palvelut/kulutuksen-ja-tuotannon-liittaminen-kantaverkkoon/specific-study-requirements-for-grid-energy-storage-systems-en.pdf (last access: May 2024), 2023. a, b
Gevorgian, V., Koralewicz, P., Shah, S., Yan, W., Wallen, R., and Mendiola, E.: Testing GFM and GFL Inverters Operating With Synchronous Condensers, in: 2023 IEEE Power & Energy Society General Meeting (PESGM), July 2023, Orlando, Florida, USA, 1–5, https://doi.org/10.1109/PESGM52003.2023.10252338, 2023. a
Ghimire, S., Amico, G., Kkuni, K. V., Guerreiro, G. M. G., Jensen, K. H., and Yang, G.: Enabling Islanding and Re-Synchronization of Grid-Forming Offshore Wind Turbine Generators, 22nd Wind and Solar Integration Workshop, October 2024, Helsinki, Finland, 2024. a
Gomes Guerreiro, G. M., Sharma, R., Martin, F., Ghimire, P., and Yang, G.: Concerning Short-Circuit Current Contribution Challenges of Large-Scale Full-Converter Based Wind Power Plants, IEEE Access, 11, 64141–64159, https://doi.org/10.1109/ACCESS.2023.3288610, 2023. a
Gomes Guerreiro, G. M., Martin, F., Dreyer, T., Yang, G., and Andresen, B.: Advancements on Grid Compliance in Wind Power: Component & Subsystem Testing, Software-/Hardware-in-the-Loop, and Digital Twins, IEEE Access, 12, 25949–25966, https://doi.org/10.1109/ACCESS.2024.3366091, 2024. a
Hans, F., Curioni, G., Jersch, T., Quistorf, G., Ruben, M., Müller, A., Wessels, C., Fenselau, C., Prima, I., and Lehmann, J.: Towards Full Electrical Certification of Wind Turbines on Test Benches – Experiences Gained from the HiL-GridCoP project, in: vol. 2022, 21st Wind & Solar Integration Workshop (WIW 2022), October 2022, the Hague, the Netherlands, 122–129, https://doi.org/10.1049/icp.2022.2746, 2022. a
Hans, F., Quistorf, G., Jersch, T., Chekavskyy, G., Bujak, G., Sobanski, P., and Eckerle, J.: Mobil-Grid-CoP – A New Approach for Grid Compliance Testing on Multimegawatt Wind Turbines in Field, in: vol. 2023, 22nd Wind & Solar Integration Workshop (WIW 2023), September 2023, Copenhagen, Denmark, 2023. a
IEA: Wind Electricity, IEA, license: CC BY 4.0, https://www.iea.org/reports/wind-electricity (last access: May 2024), 2022. a
IEA: Tracking Clean Energy Progress 2023, https://www.iea.org/reports/tracking-clean-energy-progress-2023 (last access: May 2024), 2023. a
IEC: (Ongoing) IEC CD 61400-21 Wind energy generation systems – Part 21-4: Wind turbine components and subsystems, International Electrotechnical Commission, Document 88/889/CD, https://www.vde-verlag.de/standards/1100775/e-din-iec-ts-61400-21-4-vde-v-0127-21-4-2024-04.html (last access: May 2024), 2023. a
IEEE 2800: IEEE Standard for Interconnection and Interoperability of Inverter-Based Resources (IBRs) Interconnecting with Associated Transmission Electric Power Systems, IEEE Std 2800-2022, 1–180, 2022. a
InterOPERA: Grid-Forming Functional Requirements for HVDC Converter Stations and DC-Connected PPMs in Multi-terminal Multi-vendor HVDC systems, https://interopera.eu/wp-content/uploads/files/deliverables/InterOPERA-D2.2-GFM-functional-requirements-202401.pdf (last access: May 2024), 2024. a, b, c
Johansen, K.: Grid Codes: Recommendations for Connection of Offshore Wind Power Energinet, Energinet Associated Activities, 2020. a
Kocewiak, L. H., Guest, E., Dowlatabadi, M. K. B., Chen, S., Árnadóttir, U. D., Jensen, S. J., Kramer, B. L. Ø., Nagaiceva, V., Siepker, T., and Terriche, Y.: Active filtering trial to reduce harmonic voltage distortion in an offshore wind power plant, in: vol. 2023, 22nd Wind and Solar Integration Workshop (WIW 2023), September 2023, Copenhagen, Denmark, 394–399, https://doi.org/10.1049/icp.2023.2764, 2023. a
Li, Z., Wang, X., Zhao, F., Munk-Nielsen, S., Geske, M., Grune, R., Bo Rønnest Andersen, D., and Garnelo Rodriguez, M.: Power-Hardware Design and Topologies of Converter-Based Grid Emulators for Wind Turbines, IEEE J. Em. Sel. Top. P., 11, 5001–5017, https://doi.org/10.1109/JESTPE.2023.3302808, 2023. a, b
Lyu, X. and Groß, D.: Grid Forming Fast Frequency Response for PMSG-Based Wind Turbines, IEEE T. Sustain. Energ., 15, 23–38, https://doi.org/10.1109/TSTE.2023.3263858, 2024. a
Matevosyan, J., Badrzadeh, B., Prevost, T., Quitmann, E., Ramasubramanian, D., Urdal, H., Achilles, S., MacDowell, J., Huang, S. H., Vital, V., O'Sullivan, J., and Quint, R.: Grid-forming inverters: Are they the key for high renewable penetration?, IEEE Power Energy M., 17, 89–98, 2019. a
Milano, F., Dörfler, F., Hug, G., Hill, D. J., and Verbič, G.: Foundations and Challenges of Low-Inertia Systems (Invited Paper), in: 2018 Power Systems Computation Conference (PSCC), June 2018, Dublin, Ireland, 1–25, https://doi.org/10.23919/PSCC.2018.8450880, 2018. a
Musca, R., Vasile, A., and Zizzo, G.: Grid-forming converters. A critical review of pilot projects and demonstrators, Renew. Sust. Energ. Rev., 165, 112551, https://doi.org/10.1016/j.rser.2022.112551, 2022. a
National Grid: Onshore vs. Offshore Wind Energy, https://www.nationalgrid.com/stories/energy-explained/onshore-vs-offshore-wind-energy (last access: May 2024), 2023. a
NERC: Grid Forming Technology: Bulk Power System Reliability Considerations, North Americal Electric Reliability Corporation, https://www.nerc.com/comm/RSTC_Reliability_Guidelines/White_Paper_Grid_Forming_Technology.pdf#search=Grid%20Forming%20Technology (last access: May 2024), 2021. a
NERC: White Paper: Grid Forming Functional Specifications for BPS-Connected Battery Energy Storage Systems, White Paper, https://www.nerc.com/comm/RSTC_Reliability_Guidelines/White_Paper_GFM_Functional_Specification.pdf (last access: May 2024), 2023. a
Neshati, M., Azarian, S., Curran, O., Pillai, R., Due, J., Guest, E., Freire, N., Dreyer, T., and Martin, F.: Grid Connection Testing of Wind Energy Converters with Medium Voltage Generator- and Grid Emulation on a Multi-Megawatt Power-Hardware-in-the-Loop Test Rig, in: vol. 2023, 22nd Wind & Solar Integration Workshop (WIW 2023), https://doi.org/10.1049/icp.2023.2773, 2023. a, b
NG-ESO: Stability pathfinder Oct 2019, draft grid code grid forming and request for information feedback, https://www.neso.energy/document/185306/download (last access: May 2024), 2019. a
NG-ESO: Workgroup Consultation GC0137: Minimum Specification Required for Provision of GB Grid Forming (GBGF) Capability (formerly Virtual Synchronous Machine/VSM Capability), https://www.neso.energy/document/189381/download (last access: May 2024), 2021. a, b, c, d, e, f, g
NG-ESO: Great Britain Grid Forming Best Practice Guide, https://www.nationalgrideso.com/document/278491/download (last access: May 2024), 2023. a, b, c, d
NG-ESO: System Oscillation Assessment of Inverter Based Resources (IBRs), National Grid ESO, https://www.nationalgrideso.com/document/301686/download (last access: May 2024), 2024. a
OSMOSE: D3.3 Analysis of the synchronisation capabilities of BESS power converters, https://www.osmose-h2020.eu/wp-content/uploads/2022/04/D3.3-Analysis-of-the-synchronisation-capabilities-of-BESS-power-converters.pdf (last access: May 2024), 2021. a
Pattabiraman, D., Lasseter, R. H., and Jahns, T. M.: Comparison of Grid Following and Grid Forming Control for a High Inverter Penetration Power System, in: 2018 IEEE Power & Energy Society General Meeting (PESGM), August 2018, Portland, Oregon, USA, 1–5, https://doi.org/10.1109/PESGM.2018.8586162, 2018. a
Rokrok, E., Qoria, T., Bruyere, A., Francois, B., and Guillaud, X.: Integration of a storage device to the DC bus of a grid-forming controlled HVDC interconnection, Electr. Pow. Syst. Res., 212, 108601, https://doi.org/10.1016/j.epsr.2022.108601, 2022. a
TenneT: Grid connection requirements for DC connected PPMs, System needs and functions, Annex C.2.300, https://www.tennet.eu/de/strommarkt/kunden-deutschland/netzkunden/netzanschlussregeln (last access: May 2024), 2023. a
UNIFI Consortium: UNIFI Specifications for Grid-Forming Inverter-Based Resources – Version 2, UNIFI-2024-2-1, https://www.energy.gov/sites/default/files/2023-09/Specs%20for%20GFM%20IBRs%20Version%201.pdf (last access: March 2024), 2022. a
US-EIA: Table 1.1. Net Generation by Energy Source: Total (All Sectors), https://www.eia.gov/electricity/monthly/epm_table_grapher.php?t=epmt_1_1 (last access: 18 March 2024), 2023. a
VDE FNN: FNN Guideline: Grid forming behavior of HVDC systems and DC-connected PPMs, https://www.vde-verlag.de/books/636341/grid-forming-behaviour-of-hvdc-systems-and-dc-connected-ppms.html (last access: May 2024), 2020. a
Verbe, S. C., Shigenobu, R., and Ito, M.: Comparative Study of GFM-grid and GFL-grid in Islanded Operation, in: 2021 IEEE PES Innovative Smart Grid Technologies – Asia (ISGT Asia), December 2021, Brisbane, Australia, 1–5, https://doi.org/10.1109/ISGTAsia49270.2021.9715619, 2021. a
Xu, B., Gao, C., Zhang, J., Yang, J., Xia, B., and He, Z.: A Novel DC Chopper Topology for VSC-Based Offshore Wind Farm Connection, IEEE T. Power Electr., 36, 3017–3027, https://doi.org/10.1109/TPEL.2020.3015979, 2021. a, b
- Abstract
- Introduction
- Grid-forming functional specifications
- Physical limitations of OF WPPs and classification of GFM requirements
- Testing grid-forming capabilities
- Conclusions
- Data availability
- Author contributions
- Competing interests
- Disclaimer
- Special issue statement
- Financial support
- Review statement
- References
- Abstract
- Introduction
- Grid-forming functional specifications
- Physical limitations of OF WPPs and classification of GFM requirements
- Testing grid-forming capabilities
- Conclusions
- Data availability
- Author contributions
- Competing interests
- Disclaimer
- Special issue statement
- Financial support
- Review statement
- References